Lessons in Bioreactor Scale-Up: Part 1 — Exploring Introductory Principles
February 7, 2024
Biologics such as monoclonal antibodies (mAbs), other recombinant proteins, and viral vectors now represent a major class of pharmaceuticals. Their manufacturing, based primarily on mammalian-cell culture, has advanced significantly in the past two decades. Heat and mass transfer, fluid dynamics, reaction kinetics, and other chemical-engineering principles apply broadly to biologics development and production. For cell-culture–based processes, development scientists and engineers use such principles to optimize transport of nutrients (including oxygen) to cells, mixing and removal of undesired metabolites, and collection and purification of molecules of interest.
Cell-culture process development involves investigation of both scale-dependent and scale-independent bioreactor parameters. Scale-independent parameters — e.g., pH, temperature, dissolved oxygen (DO) concentration, and media composition and osmolality — typically are tested and optimized in small-scale bioreactors, then kept constant during scale-up. Scale-dependent parameters, on the other hand, are affected by a bioreactor’s geometric configuration and operating parameters. Bioreactor-impeller rotational speed (N), gas-sparging rates, working volume, and so on affect the state of fluid flow and mixing (homogenization) in a bioreactor, thus influencing the physical forces that act on cells. Hence, bioreactor scale-up requires optimization of operating parameters for the large-scale bioreactor to be used in a production process.
Bioreactor scale-up influences physical, chemical, and biological factors of cell culture. Physical factors, as mentioned above, include bioreactor configuration, aeration, agitation, heat transfer/removal, and mixing. Chemical factors affected by scale-up include type and concentration of acid/base needed for pH control, water quality, and foam formation caused by surface tension changes. Biological factors include the number of generations associated with inoculum development and production phases, mutation probability, contamination vulnerability, and elimination of selection pressure (1).
A biologic manufacturing process typically involves scaling up of production from miniaturized, high-throughput bioreactors (15–250 mL) to bench-scale glass or single-use reactors (1–10 L) and then pilot- and production-scale bioreactors (200–5,000 L or larger). Bioreactor scaling is not trivial; it is a difficult and complex task that requires a delicate balance between equipment design and operational capabilities, which often vary considerably across scales, to provide similar hydrodynamic and mass-transport conditions for cell growth and production. Several factors contribute to the difficulty of scaling. They include the complexity of biological systems and the heterogeneous (hydrodynamic and mass-transfer) environments prevailing in large-scale bioreactors leading to substrate and pH gradients. Such complexity leads to variations in cell growth, metabolism, protein production, and sometimes, product-quality profiles across scales — commonly known as process variability due to bioreactor scaling.
Because single-use bioreactors are now commonplace, many contract development and manufacturing organizations (CDMOs) and other commercial manufacturers often have production bioreactors located at many manufacturing sites across a country or even around the globe. Those bioreactors can differ not only in their geometric configurations — e.g., in their bioreactor height:tank diameter (H/T) and impeller diameter:tank diameter (D/T) ratios — but also in their sparger configurations and impeller types. Many suppliers of single-use bioreactors, however, now provide a “family” of bioreactors with geometrically similar designs at working volumes that span from development (10–50 L) to pilot and production scales (200–2,000 L).
Key Considerations in Bioreactor Scale-Up
Key factors encountered during bioreactor scale-up include
• nonlinearity
• differences in (bio)chemical equilibrium
• differences in fluid dynamics and agitation settings
• development of temperature, oxygen, pH, and CO2 gradients
• material properties and equipment selection.
Nonlinearity in Bioreactor Scaling: Geometric similarity — maintaining similar H/T and D/T ratios across bioreactor sizes — is often a prerequisite for scale-up (Figure 1). To accomplish that, all length dimensions are scaled proportionally. Laboratory-scale bioreactors often have an H/T ratio of 2/1. Large-scale bioreactors, on the other hand, can range in ratio from 2/1 to 4/1. Meanwhile, D/T ratios generally are maintained in the range of 1/3 to 1/2.
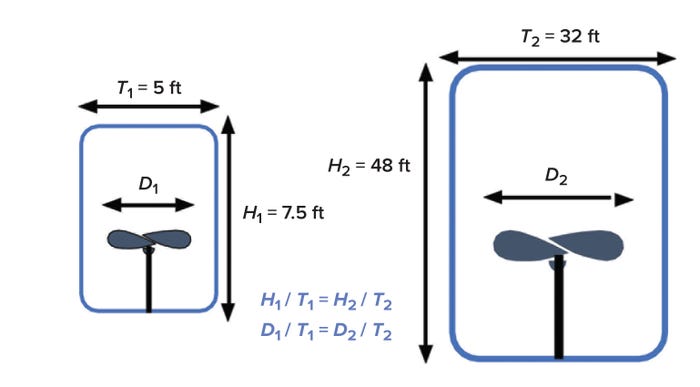
Figure 1: Bioreactor geometric similarity and change in volume at a constant scale-up factor; D = impeller diameter, H = bioreactor height, T = tank diameter, 1 = small-scale vessel, 2 = large-scale vessel.
One consequence of holding H/T ratios constant during scale-up is a dramatic reduction in the ratio of surface area to volume (SA/V) (Figure 2) (2). Doing so also increases bioreactor volume dramatically. As Figure 1 illustrates, maintaining an H/T value of 1.5 and using a scale-up factor of 6.4 changes the volume from 147 ft3 in the small bioreactor to 38,604 ft3 at the larger scale — a 26-fold increase.
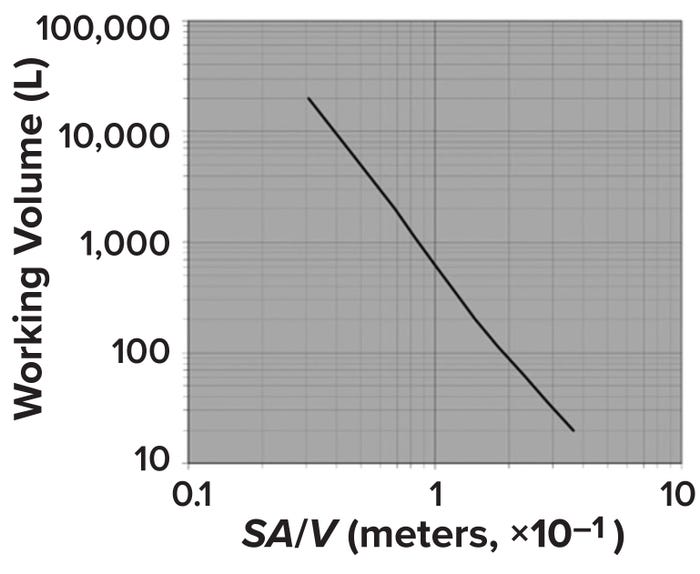
Figure 2: Changes in surface area per unit volume (SA/V) with increase in bioreactor size; adapted from Marks (2).
The resulting decrease in SA/V ratio creates challenges for heat removal in large-scale microbial fermenters. For animal-cell–culture bioreactors, CO2 removal becomes an issue at large scales because of the increase in bioreactor height, decrease in liquid surface area at the top, and added head pressure from the higher liquid height. All those factors decrease the efficiency of CO2 stripping in the bioreactor overlay. Scale-up generally results in higher shear-force variation with less efficient bulk mixing because of longer circulation times and larger stagnant areas.
Many other parameters change during scale-up, such as a transition from laminar to turbulent flow regimes. Table 1 shows interdependence among several scale-up criteria based on a scale-up factor of 125. It is evident from the table that the bigger the scale-up factor is, the more that the process parameters — and scale-up criteria — change. An analysis of those data makes it clear that even if geometric similarity (H/T) is maintained during scale-up, then conditions in a large-scale bioreactor never will exactly duplicate those in a small-scale bioreactor because of changes in parameter values.
Table 1: Interdependence of scale-up parameters; the “scale-up criterion” column indicates which variable is held constant between two scales. Table contents are adapted from Lara et al. (4).
Adequate mixing in large-scale bioreactors generally requires an extremely high power input, which is infeasible either because of equipment-size and mechanical constraints or shear sensitivity in mammalian cells. Consequently, substrate and pH gradients generally occur in large-scale bioreactors. Both product yields and product-quality profiles can be different in large-scale bioreactors.
In addition to maintaining H/T ratios, process scientists must determine other design parameters to be within acceptable ranges using the ratios of the impeller diameter (D), impeller-bottom clearance, liquid height, vessel height, and baffle width to the vessel diameter. Traditionally, one of the following scale-up criteria or combinations thereof have been used in microbial and animal-cell culture scale-up:
• a constant impeller-tip speed
• a constant power per unit volume (P/V)
• a constant oxygen mass-transfer coefficient (kLa)
• a constant mixing time
• a constant Reynold’s number (Re)
• a constant gas-flow rate per unit volume (vvm)
• a combination of these criteria, such as constant P/V and vvm or a constant kLa and gas-flow rate (or gas velocity) across scales (3).
Table 1 lists parameters considered during scale-up, all of which depend on tank diameter and impeller rotational speed (D and N values, respectively). Fixing those values also fixes all other quantities used in scale-up calculations. Because power input, impeller-tip speed, Reynold’s number, and the like have different dependencies on D and N values, a change in those numbers (due to a change in scale) also will alter the physical environment in which cells are cultured. Such changes can affect the distribution of chemical species and, in extreme cases, injure or kill cells. In addition, cellular metabolic responses can change from one scale to another, which ultimately would result in a change in cell physiology.
It is clear from Table 1 that scaling up when maintaining equal P/V ratios results in lower impeller speeds but higher tip speeds, longer circulation times, and greater kLa values. On the other hand, scale-up based on impeller speed (N) is not feasible because doing so would decrease P/V ratios substantially. Scale-up based on equal Re values is also infeasible because P/V would be reduced by a factor of 625.
Circulation time — the average time that a particle requires to travel from and return to a fixed location in the bioreactor, a parameter that is directly proportional to mixing time — generally is not used as a primary scale-up criterion. Except when using equal impeller rotation speeds (N) across bioreactors, all other scale-up criteria increase circulation time. Holding circulation time constant results in a 25-fold increase in P/V. Similarly, scale-up based on equal tip speed reduces P/V ratios by a factor of 5 and also would result in lower kLa values. In addition, circulation time increases fivefold.
In summary, the scale-up issues discussed above all relate to transport phenomena: heat, mass, and momentum transfer. Scale-up generally involves moving processes being controlled by cell kinetics at laboratory scale to control by transport limitations at larger scales. Consequently, time scales for mixing and reaction rates become important in determining the level of homogeneity in a large-scale bioreactor. Therefore, the objective of a scale-up exercise is not to keep scale-dependent parameters constant; rather, it is to define the operating range(s) of scale-sensitive parameters such that cellular physiological states — and thus cell-line productivity and product-quality profiles — can be maintained across scales.
Biochemistry and Chemical Equilibrium: Most chemical reactions, including cellular metabolism, require good mixing to have uniform reactant and/or substrate concentrations. Such processes also can release heat as components react to form products. Cellular — and especially microbial — metabolism generates heat that must be removed from a bioreactor to maintain constant temperature. Changes in SA/V ratio upon scale-up can influence heat-transfer efficiency and, in turn, affect how reactions proceed inside a large bioreactor. Cells generally prefer narrow ranges in temperature, pH, osmolality, and substrate concentrations for optimal performance. Hence, large-scale processes must be optimized to provide the best possible environment for cell growth and biologic production.
Mixing, Agitation, and Fluid Dynamics: As discussed above and as shown in Table 1, scale-up based on equal P/V values increases circulation time (and hence mixing time) by almost threefold. Consequently, environmental heterogeneities such as substrate, pH, and oxygen gradients can occur in large-scale bioreactors. As cells travel through such gradients, they will be exposed to a continually changing environment, which can alter overall culture performance. Mixing times are higher in animal-cell bioreactors than in microbial fermenters because, in the former case, low to moderate power input must be applied. In large-scale cell-culture bioreactors, mixing times can be in the order of minutes (4).
Fluid dynamics change nonlinearly as bioreactor size increases. Hence, transitions from laminar- to turbulent-flow conditions occur, and generally such changes are difficult to predict. Keeping flow at the correct Re value (which helps to predict laminar- and turbulent-flow conditions) enables a culture process to maintain thermal and mixing efficiency. The type of mixing regime needed for a given process depends on whether cells are adherent or flow freely in suspension. During scale-up, Re values often change based on other scale-up criteria; hence, a mixing regime can change from laboratory to clinical and commercial scales, complicating successful scale-up.
Development of Gradients in Large-Scale Bioreactors: Low power input to large-scale cell-culture bioreactors generally results in longer mixing times, which can cause stagnant zones to develop. While circulating in a bioreactor, cells therefore can experience transient changes in environmental parameters such as pH levels and oxygen concentrations. Such transient excursions in a bioreactor environment can influence cellular physiology. By-products of mammalian-cell metabolism (e.g., lactate) accumulate over the culture period and, if mixing is inadequate, will generate zones of low pH in the bioreactor. Similarly, a transient increase in metabolic activity can cause oxygen depletion in high-nutrient concentration zones.
To summarize, bioreactor parameters interact in complex ways because of heterogeneities in large-scale vessels, and a gradient in one culture variable can originate gradients in other variables (4). Such effects can alter cell phenotypes or diminish genetic stability of the plasmid or vector containing a recombinant gene of interest.
Oxygen is sparingly soluble in water and hence must be supplied continuously during cell culture. Mixing times (in the order of minutes) can spur on development of oxygen gradients in large-scale bioreactors. Lara et al. postulate that, in a mammalian-cell stirred-tank bioreactor of 20 L, oxygen gradients can occur if cell concentrations exceed 20 million cells/mL of culture fluid (4). Such concentrations are relatively common in large-scale bioreactors operated in fed-batch mode.
Because of the dynamic nature of mammalian-cell metabolism in stirred-tank reactors, operated either in a batch or fed-batch mode, culture pH decreases over time due to accumulation of acidic by-products. Bioreactor pH, therefore, needs to be controlled by the addition of a base. But poor mixing could give rise to a high-pH zone near the base addition port. The pH level in such zones can rise transiently to nonphysiological values, affecting not only cellular metabolism, but also intracellular pH, changes in which can affect enzyme activity. For example, a change in intracellular pH can alter glucose transport, ratios of adenosine triphosphate (ATP) to adenosine diphosphate (ADP), and product-quality profiles.
During a mammalian-cell fed-batch culture, the increase in biomass concentration over time results in production of CO2 as a by-product of metabolism. Compared with oxygen, CO2 has higher solubility in culture media, and depending on culture pH, it can be absorbed as dissolved CO2 (dCO2). In an ideal situation, depending on air-sparging rates and bubble sizes, most dCO2 is stripped by air and is removed from the bioreactor as part of the exit-gas stream. However, the decrease in SA/V ratio during scale-up diminishes gas exchange on the liquid surface and hampers dCO2 stripping by air flowing through the overlay or headspace. Thus, in large-scale bioreactors, dCO2 is higher than it is in laboratory-scale vessels because the increased liquid column height also increases head (hydrostatic) pressure. Heightened hydrostatic pressure at the bottom of a bioreactor increases gas solubility and results in accumulation of dissolved gases (1).
Accumulation of dCO2 has several effects on culture conditions, including changes in culture pH due to CO2 being in equilibrium with sodium bicarbonate in the medium. That generates carbonate and bicarbonate ions, both of which decrease culture pH, thereby changing intracellular pH (5–8). Such ions also increase osmolality at constant bioreactor pH (9). Gray et al. report that air bubbles of 2–3 mm and a sparging rate of 0.005 vvm are required to operate a 500-L Chinese hamster ovary (CHO)–cell perfusion bioreactor without oxygen limitation and dCO2 accumulation (10).
Material Properties and Equipment Selection: Physical and/or chemical properties of the material of construction used in small- and large-scale bioreactors can influence not only a culture’s progress, but also its outcomes. Single-use and high-throughput bioreactors have become commonplace for laboratory-scale experimentation. Now, many commercial-scale cell-culture processes are carried out in single-use bioreactors of different sizes. Commercial-scale processes developed in the 1990s were performed in stainless-steel bioreactors of multiple-thousand liters in scale. For such equipment, cleaning validation is a requirement. Because of improvements in cell-culture media and bioprocess optimization over the past two decades, most modern commercial processes are performed at 2,000-L scale.
How To Scale Up — The Role of Process Parameters
Process engineers and development scientists can perform bioreactor scale-up using either agitation- or gassing-based parameters.
Mixing time, P/V ratio, and impeller-tip speed are parameters that fall under the category of agitation-based scaling parameters. Because those parameters depend on agitation, they cannot all be held constant during scaling. Keeping mixing time constant generally necessitates very high P/V values, whereas keeping tip speed constant might require low agitation rates that could create oxygen-delivery issues. Hence those parameters need to be evaluated individually, and final scale-up agitation must result in acceptable values for all three.
Power input per unit volume (P/V) refers to the amount of power transferred by the agitator motor through the agitator shaft and impellers to a given volume of cell-culture broth. P/V is calculated using Equation 1:
P/V = P0 ρ N3 D2
Therein, P0 represents the impeller power number, N is the impeller speed, D is impeller diameter, and ρ denotes the liquid density. To scale up based on maintaining P/V constant across scales implies Equation 2,
(P0 ρ N3 D2)2 = (P0 ρ N3 D2)1
in which subscripts 2 and 1 refer to large- and small-scale bioreactors, respectively.
Liquid density (ρ) can be held constant if a culture medium will not change during scale-up. The impeller power number (P0) also can be considered constant when preserving geometric similarity across scales, assuming fully developed turbulent flow, and using the same impeller type, configuration, and spacing.
Equation 2 can be simplified to Equation 3:
(N3 x D2)2 = (N3 x D2)1
And large-scale rotational speed (agitation) can be calculated using Equation 4:
N2 = N1 x (D1 D2)2/3
As that equation shows, at large scales, the agitation rate must be decreased proportionally to the impeller diameter ratio to 2/3 power.
Impeller-Tip Speed: Tip speed serves as a measurement of the shear rate produced by impeller blades in a cell-culture medium. Using tip speed as a primary scale-up criterion generally leads to a decrease in P/V and hence is used infrequently for animal cell culture. However, Equation 5 can be used to calculate the impeller rotation speed (agitation) at the large scale:
π x D2 x N2 = π D1 x N1
That can be rearranged to Equation 6,
N2 = N1 x (D1 ÷ D2)
The latter equation shows that, at the large scale, agitation must be decreased proportionally to the impeller diameter.
Mixing time represents how much time is needed for bioreactor content to attain a homogeneous state. The parameter depends on both the level of turbulence within and bulk turnover of that content. Mixing times on the order of 160 seconds have been reported for large-scale (100 m3) fermenters (1). Inefficient mixing in a large cell-culture bioreactor can generate pH, oxygen, and substrate gradients. Mixing time also depends on impeller speed (agitation rate), impeller type and size, impeller spacing from the bottom of the vessel, and baffle design (if applicable). When using equal mixing time as the primary scale-up criterion, Equation 7 can be used to determine the impeller rotation speed for the large-scale bioreactor (with 2 and 1 again representing large and small vessels):
N2 = N1 x (D1 ÷ D2)1/4
Again, using equal mixing time, agitation in a large-scale reactor must be decreased proportionally to the impeller diameter to the power of 1/4. Because scale-up based on equal mixing time results in large P/V values, it is used infrequently as a scale-up criterion for mammalian-cell bioreactors.
The above analysis shows that it is not possible to keep all the discussed scaling parameters constant. Scale-up still is performed based on industry experience, knowledge gained during process development, and when available, familiarity with the historical performance of a target bioreactor.
Gassing-Based Parameters: During bioreactor scale-up, liquid height:tank diameter ratios often can be >1 for the larger vessel, limiting back-mixing of upward-moving sparged gas. Consequently, vertical DO gradients will occur if bulk mixing is slower than the oxygen mass-transfer rate, and radial DO gradients will emerge because shear rates decline rapidly with increased distance from the impeller (1). Hence, in large-scale bioreactors, the most important — and most difficult — aspect of a scale-up exercise often is providing sufficient oxygen to the culture while maintaining an oxygen-transfer rate (OTR) equivalent to that of the small-scale vessel. Successful scale-up based on providing a constant OTR depends on the ability to measure or estimate kLa values and the gassed power per unit liquid volume (Pg/V).
Scale-up based on a constant OTR assumes that the oxygen uptake rate (OUR) in a bioreactor equals the OTR, as shown in Equations 8 and 9:
OTR = kLa (Csat – CL)
OUR = μ x X x YX/O2
Therein, Csat denotes the bioreactor DO concentration at saturation, CL represents the measured DO concentration (at any time), μ is the specific growth rate, X is the measured cell density, and YX/O2 is the yield coefficient (the calculated cell yield per amount of oxygen consumed).
Changes in back-pressure and hydrostatic pressure will influence Csat and subsequently CL values. In tall bioreactors, local and saturation concentrations of oxygen can differ at the top and bottom of the vessel. To account for such differences, the log mean of the DO concentration difference should be used. Because the critical DO concentration that adversely affects growth rate is low in most aerobic cell cultures, CL is assumed to be zero.
Available literature provides several empirical correlations of the general form (Equation 10)
kLa = C(Pg/V)α x Vsβ
to estimate kLa using gassed power per unit volume (Pg/V) and gas superficial velocity (Vs). Values for the constant (C) and exponents (α and β) are process-specific, and a broad range of values have been reported. Generally, the dependence of kLa on Pg/V decreases with increase in scale.
Such correlations have been reported for multiple gas–liquid systems and bioreactors (11). However, exercise caution when using those correlations for bioreactor scale-up because doing so can generate significant errors.
Although both kLa and CL can vary substantially during a fed-batch run, the minimum acceptable value of CL is generally known (based on experiments in laboratory-scale bioreactors). A CL value >30–70% saturation generally ensures adequate DO concentrations in less-mixed regions of a bioreactor (1). That said, scale-up based on maintaining constant DO across scales can be tricky, and engineers and development scientists must consider methods to control DO such as agitation, air and oxygen flow rates, air enrichment with oxygen, and even use of pure oxygen. Cascade control using such methods is generally effective in maintaining DO above critical values.
Maintaining kLa with either equal vvm (volumetric gas-flow rates) or equal Vs (superficial gas velocity) across scales is a common scale-up principle for mammalian-cell bioreactors. Both principles result in similar P/V values across scales and have been applied successfully in commercial production processes. When basing scale-up on vvm, Vs values also should be calculated and checked to ensure that resulting values are reasonable.
A process must balance vvm and Vs values. If vvm is kept constant, then Vs can increase to the point of flooding the impeller in a production-scale bioreactor (1). If it is advantageous to hold Vs constant during scale-up, the vvm value should be larger at the smaller scale than at the target scale. Although vvm has little effect on the value of kLa, higher air-flow rates can increase foaming dramatically and increase gas hold-up. If both Pg/V and Vs are kept constant during scale-up, then gas hold-up does not change with scale-up.
Single-use bioreactors often are designed with unique (proprietary) impellers. Those can be bottom-mounted or attached using a shaft with an offset from the vessel center to improve mixing. In such instances, experimental data must be collected to identify P/V values and gas-flow rates that will meet target kLa values — and thus satisfy oxygen demands and ensure solution homogeneity.
In large-scale bioreactors, air sparging is used both for oxygenation and CO2 stripping. Whereas oxygen transfer depends on superficial gas velocity, CO2 removal is dependent on total gas-flow rates (vvm). Therefore, scaling based on vvm values can result in inadequate CO2 stripping. That is generally a concern for bioreactors of >2,000 L. Xu et al. discuss strategies for ensuring proper oxygenation and CO2 removal (12).
Continued Exploration
This article represents the first in a series of explorations into bioreactor scale-up. Read the April 2024 issue of BPI to find the next installment, which will overview fundamental aspects of fluid flow and mixing in bioreactors.
References
1 Junker BH. Scale-Up Methodologies for E. coli and Yeast Fermentation Processes. J. Biosci. Bioeng. 97(6) 2004: 347–364; https://doi.org/10.1016/s1389-1723(04)70218-2.
2 Marks DM. Equipment Design Considerations for Large-Scale Cell Culture. Cytotechnol. 42(1) 2003: 21–33; https://doi.org/10.1023/a:1026103405618.
3 Alavijeh MK, et al. Digitally Enabled Approaches for the Scale-Up of Mammalian Cell Bioreactors. Dig. Chem. Eng. 4, 2022: 100040; https://doi.org/10.1016/j.dche.2022.100040.
4 Lara AR, et al. Living with Heterogeneities in Bioreactors. Molec. Biotechnol. 34(3) 2006: 355–381; https://doi.org/10.1385/mb:34:3:355.
5 Mostafa SS, Gu XS. Strategies for Improved dCO2 Removal in Large-Scale Fed-Batch Cultures. Biotechnol. Prog. 19(1) 2003: 45–51; https://doi.org/10.1021/bp0256263.
6 Zanghi JA, et al. Bicarbonate Concentration and Osmolality Are Key Determinants in the Inhibition of CHO Cell Polysialylation Under Elevated pCO(2) or pH. Biotechnol. Bioeng. 65(2) 1999: 182–191; https://doi.org/10.1002/(SICI)1097-0290(19991020)65:2%3C182::AID-BIT8%3E3.0.CO;2-D.
7 Mitchell-Logean C, Murhammer DW. Bioreactor Headspace Purging Reduces Dissolved Carbon Dioxide Accumulation in Insect Cell Cultures and Enhances Cell Growth. Biotechnol. Prog. 13(6) 2008: 875–877; https://dx.doi.org/10.1021/bp970078s.
8 Garnier A, et al. Dissolved Carbon Dioxide Accumulation in a Large Scale and High Density Production of TGFβ Receptor with Baculovirus Infected Sf-9 Cells. Cytotechnol. 22(1–3) 1996: 53–63; https://doi.org/10.1007/bf00353924.
9 de Zengotita VM, Kimura R, Miller WM. Effects of CO2 and Osmolality on Hybridoma Cells: Growth, Metabolism and Monoclonal Antibody Production. Cytotechnol. 28, 1998: 213–227; https://doi.org/10.1023/a:1008010605287.
10 Gray DR, et al. CO2 in Large-Scale and High-Density CHO Cell Perfusion Culture. Cytotechnol. 22(1–3) 1996: 65–78; https://doi.org/10.1007/bf00353925.
11 Garcia-Ochoa F, Gomez E. Bioreactor Scale-Up and Oxygen Transfer Rate in Microbial Processes: An Overview. Biotechnol. Adv. 27(2) 2009: 153–176; https://doi.org/10.1016/j.biotechadv.2008.10.006.
12 Xu P, et al. Characterization of TAP Ambr 250 Disposable Bioreactors as a Reliable Scale-Down Model for Biologics Process Development. Biotechnol Prog. 33(2) 2017: 478–489; https://doi.org/10.1002/btpr.2417.
Further Reading
Chu L, Robinson DK. Industrial Choices for Protein Production By Large-Scale Cell Culture. Curr. Opin. Biotechnol. 12(2) 2001: 180–187; https://doi.org/10.1016/s0958-1669(00)00197-x.
Malik P, Mukherjee TK. Large-Scale Culture of Mammalian Cells for Various Industrial Purposes. Practical Approach to Mammalian Cell and Organ Culture. Mukherjee TK, Malik P, Mukherjee S, Eds. Springer Singapore: Singapore, 2023, 1–45; https://doi.org/10.1007/978-981-19-1731-8_15-1.
Paul K, Herwig C. Scale-Down Simulators for Mammalian Cell Culture as Tools To Access the Impact of Inhomogeneities Occurring in Large-Scale Bioreactors. Eng. Life Sci. 20(5–6) 2020: 197–204; https://doi.org/10.1002/elsc.201900162.
Schmidt FR. Optimization and Scale Up of Industrial Fermentation Processes. App Microbiol. Biotechnol. 68(4) 2005: 425–435; https://doi.org/10.1007/s00253-005-0003-0.
Szkodny AC, Lee KH. Biopharmaceutical Manufacturing: Historical Perspectives and Future Directions. Annu. Rev. Chem. Biomolec. Eng. 13, 2022: 141–165; https://doi.org/10.1146/annurev-chembioeng-092220-125832.
Varley J, Birch J. Reactor Design for Large Scale Suspension Animal Cell Culture. Cytotechnol. 29, 1999: 177–205; https://doi.org/10.1023/a:1008008021481.
Xing Z, et al. Scale-Up Analysis for a CHO Cell Culture Process in Large-Scale Bioreactors. Biotechnol. Bioeng. 103(4) 2009: 733–746; https://doi.org/10.1002/bit.22287.
Zakrzewski R, Lee K, Lye GJ. Development of a Miniature Bioreactor Model To Study the Impact of pH and DOT Fluctuations on CHO Cell Culture Performance as a Tool to Understanding Heterogeneity Effects at Large-Scale. Biotechnol. Prog. 38(4) 2022: e3264; https://doi.org/10.1002/btpr.3264.
A seasoned industry professional with a PhD in chemical and biological engineering from the University of British Columbia (Vancouver, Canada) and expertise in embryonic stem cell bioengineering, upstream biological process development, and fed-batch bioreactors, Muhammad Arshad Chaudhry works as associate director of process and formulation development at Mural Oncology, 852 Winter Street, Waltham, MA 02451; [email protected].
You May Also Like