The Emerging Generation of Chromatography Tools for Virus Purification
October 1, 2008
Chromatography media and methods have evolved continuously since their introduction a half century ago. Traditional methods use columns packed with porous particles. They still dominate chromatography applications in the field of virus purification, but the past 20 years have witnessed the ascendance of alternative supports, namely membranes and monoliths. These newer media exploit the familiar surface chemistries — ion exchange, hydrophobic interaction, and affinity — but they use unique architectures that offer compelling performance features.
The Architecture of Chromatography Media
A monolith can be defined as a continuous stationary phase cast as a homogeneous column in a single piece (1,2,3). Monoliths are further characterized by a highly interconnected network of channels with sizes ranging 1–5 µm. The adsorptive surface is directly accessible to solutes as they pass through the column. The current generation of preparative monoliths have bed heights ranging from a few millimeters to a few centimeters. Strictly speaking, membranes are monoliths. Their channel diameters similarly range from about 0.5 to a few micrometers, but their “bed heights” are fractions of a millimeter. Traditional chromatography media are prepared as porous particles and packed in columns. Most of their adsorptive surface area resides within shallow dead-end pores ranging 50–100 nm in size (4). Figure 1 compares the generalized structures of membranes, monoliths, and porous particles.
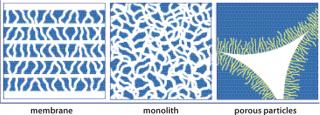
Figure 1: ()
Mass Transport
Membranes and monoliths differ most fundamentally from porous particle media in the mechanisms by which solutes are carried to and from their surfaces. The two primary modes of mass transport are diffusion and convection.
Diffusion can be defined as the migration of solutes from an area of high concentration to an area of low concentration by incremental random thermal movement. Porous particle-based media rely almost exclusively on diffusive mass transport (4). Table 1 lists diffusion constants for representative solutes. Two important points emerge: Diffusion is slow, and it becomes dramatically slower with increasing molecular size. As a direct consequence, dynamic binding capacity on porous particles decreases with increasing flow rates — dramatically so for large solutes such as viral particles (1,2,3,4,5,6,7). Resolution also suffers, also in proportion to flow rate, and also more with large solutes.
Table 1: Diffusion constants for selected solutes
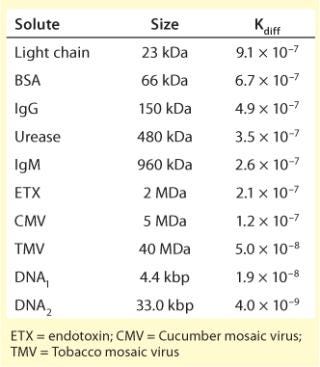
Table 1: Diffusion constants for selected solutes ()
Diffusive limitations on porous particles can be compensated somewhat by reducing flow rate, but the diffusion constants of viral particles may be an order of magnitude slower than proteins, and few industrial users are willing to reduce flow rates to that degree (Table 1). Capacity and fractionation performance are thereby compromised. Pore exclusion is an additional limitation (8). Figure 2 illustrates a scale comparison of MVM and MuLV in relation to 100-nm diffusive pores and 1-µm convective channels. Pore size is roughly five times greater than the diameter of MVM, but MuLV is excluded and has access only to the external particle surface. This severely truncates binding capacity for large viral particles. Table 2 shows that many viral species have diameters above the exclusion limits of most porous particle-based media, but surface access within the monolith is unrestricted.
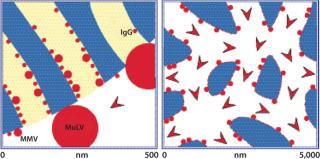
Figure 2: ()
Table 2: Approximate diameters of selected viral particles
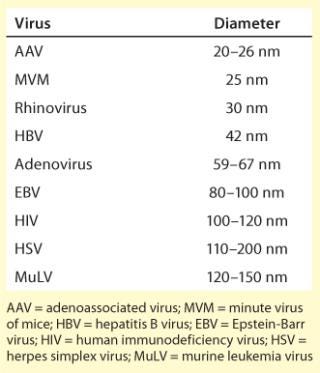
Table 2: Approximate diameters of selected viral particles ()
Convection is movement imposed by an external force, in this case fluid flow delivered by the pumps in a chromatography system. Convective mass transport is not limited by diffusion or by molecular size. A wine cork and a tree trunk both flow down a river at the same rate, which is determined by the velocity of the current. The architecture of membranes and monoliths is designed specifically to take advantage of convective mass transport. Capacity and resolution are largely independent of flow rate, even at velocities 10–20 times faster than are commonly used with diffusive particles (1,2,3, 5,6,7).
High flow rates offer numerous practical benefits. Process development and validation are accelerated, and manufacturing productivity is increased. Short process times may also be beneficial for live and attenuated viruses that are labile under the conditions used to conduct a particular purification step (8, 9). Figure 3 compares the dynamic binding capacity of a common particle-based anion exchanger with that of a monolithic anion exchanger. DNA is used as a surrogate to illustrate the behavior of large solutes. Capacity on the monolith is 30–50 times higher (10), which allows for smaller columns and translates into proportional savings in media, buffer consumption, and use of expensive manufacturing space.
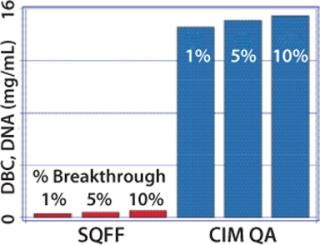
Figure 3: ()
Void Contributions
Another key distinction between particles and monoliths is the presence or absence of an interparticle void volume. Fluid takes the path of least resistance through a bed of packed particles, that is, through the void volume rather than through the particles. That disfavors solute contact with chromatography surfaces and contributes to lower virus binding capacity.
Also, fluid friction at the particle surfaces causes formation of eddies (vortices that cause turbulent mixing, dilute peaks, and erode resolution). Void space constitutes about 40% of total column volume, so it should be no surprise that eddy dispersion is a major cause of peak broadening in packed particle beds (7, 11, 12). As Figure 4 shows, eddies also create shear forces that can damage labile molecules (11,12,13). Eddy dispersion remains constant with increasing flow rate, but shear increases in direct proportion.
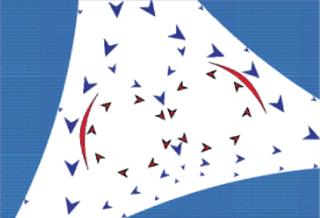
Figure 4: ()
Flow is laminar through monoliths; no eddies are formed (4, 7). In addition to minimizing shear, this assures an instantaneous response to changes in buffer composition, which maximizes elution kinetics and contributes to sharper, better resolved, and more concentrated elution peaks (14).
Flow Distribution within Columns and Housings
Although membranes lack an interparticle void, flow distribution within membrane housings is less well controlled than with either monoliths or packed particle columns, and the resulting flow aberrations compromise performance. Uncontrolled flow distribution within a housing on the inlet side of the membrane reduces capture efficiency (15). Figures 5 and 6 illustrates this effect. Figure 6 compares dynamic break-through curves for DNA on membrane and monolithic anion exchangers. Break-through is sharp on the monolith but relatively gradual on the membrane, indicating less efficient uptake. In addition, the membrane’s binding capacity is only about a third that of the monolith (10).
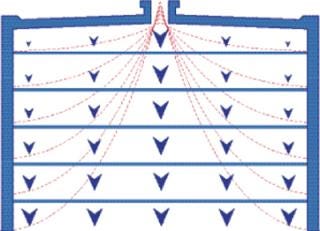
Figure 5: ()
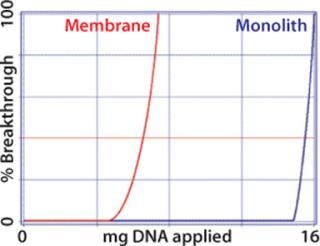
Figure 6: ()
Uncontrolled mixing on the outlet side of the membrane creates dispersion, which also diminishes overall performance. This is unlikely to be a major concern for applications in which viral particles flow through while contaminants are retained (Figure 7), but it reduces resolution in bind–elute applications (Figure 8) and may prevent effective separation between a product and contaminants with similar binding characteristics, such as full and empty capsids (16). Uncontrolled flow within membrane housings may also create eddies with their accompanying risk of shear. Shear can be reduced by lowering the flow rate, but this does not reduce dispersion, and it compromises productivity.
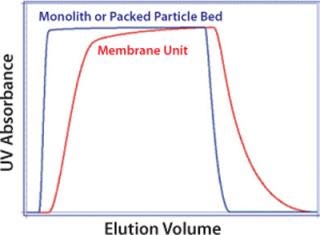
Figure 7: ()
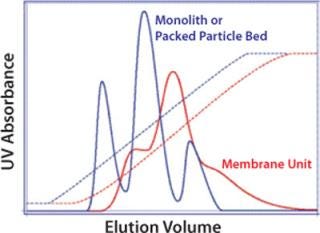
Figure 8: ()
Column designs for porous particles and monoliths are optimized to eliminate areas of uncontrolled dispersion and to provide even flow distribution at the column entrance and exit. For all chromatography supports, consideration must be given to dispersion and shear from sources external to the separation media (e.g., pumps, tubing, connectors, bubble traps, and mixers), all of which have been shown to significantly affect purification performance and product quality (17, 18).
Other Practical Distinctions
Introduction of air into a column of packed particles usually destroys the bed integrity, requires column repacking, and may require reprocessing or disposal of the material being processed at the time of the incursion. Neither monoliths nor membranes require packing to begin with, and air does not disrupt their structure. Between the two, however, monoliths are more tolerant. They use the same highly wettable polymers that are used to produce many particle-based media. Restoration of fluid flow displaces air from the channels so normal operation can resume (19). Some membranes use more hydrophobic polymers that resist rewetting. In addition, their housings allow accumulation of bubbles that may be difficult to displace without breaking system sterility.
Operating pressures at a given flow rate are lowest on membrane adsorbers and usually highest on porous particle supports. Monoliths create slightly less backpressure than packed-bed columns at flow rates lower than one column volume (CV) per minute. Monolith operating pressures increase modestly at flow rates of 5–10 CV/min but remain well within the operating ranges of popular process development and industrial chromatographs, even for high-viscosity solutions such as 10% polyethylene glycol (PEG).
Most membrane adsorbers are designed for single-use applications, which saves the expense of developing and validating cleaning and sanitization procedures for them. Monolith are moving in the same direction, but it is unclear whether packed particle media will be able to follow the disposables trend.
Harmonizing the Old with the New
Size-exclusion chromatography (SEC) exhibits the slowest flow rates and lowest capacities of all the particle-based methods. This imposes a burden on manufacturing productivity but SEC is one of the few particle-based methods without a higher-performance convective equivalent. Several valuable features compensate for its limitations: It removes the bulk of protein and small-molecule contaminants; it elutes viral particles in whatever buffer was used to equilibrate the column; and its usually gentle operating conditions generally help to conserve viral viability despite long fractionation times (8, 20,21,22,23,24,25,26). These features make SEC a valuable complement to adsorptive methods.
Fractionation is accomplished by solute transport into and out of the pores of SEC media and through the void channels among particles as a sample passes down a column. SEC media particles have a defined pore size distribution. Small solute molecules can diffuse into all the pores. They travel a long cumulative path length, which corresponds to a long residence time, and they elute at the end of the chromatogram. Progressively larger molecules can access a smaller subset of pores, which corresponds to a shorter cumulative path length, shorter residence time, and earlier elution. Molecules too large to enter any pores pass through the interparticle void volume, the shortest path through the column, and shortest residence time, so they elute at the beginning of the chromatogram.
Most viral applications use SEC media that were designed for protein fractionation. Proteins and small-molecule contaminants diffuse into the pores and elute slowly, but viral particles are excluded and pass quickly through the void volume. Having virus transport restricted to the void volume liberates viral particles from diffusive mass transport and enables effective fractionation at flow rates of 50–100 cm/hr. Higher flow rates may be considered but only with due vigilance for the effects of shear. Void transport also suspends the need to use tall, narrow columns. Ten- to 20-cm bed heights can be used with wide-diameter beds that further reduce process time. In addition, sample volumes can be increased, commonly to 20% CV and sometimes up to 30%, depending on the contaminant removal abilities of the other steps in a process.
Dramatic Advantages
Membranes and monoliths have already demonstrated unique practical value, and they are gathering momentum in the industrial purification of viral particles (8, 21, 27,28,29,30,31,32). Porous particle applications can be expected to persist — especially in the case of SEC, which offers capabilities that cannot be reproduced by convective media. Porous particles also offer a wider diversity of adsorptive surface chemistries than convective alternatives do. This has proven to be an asset to the field of industrial protein purification in which, for example, the broad selection of particle-based ion exchangers allows process developers to customize their properties to the needs of a particular application. The range of ligands available on membrane adsorbers remains limited by comparison, but industrial monoliths offer a good and growing selection of ion exchangers as well as hydrophobic interaction, immobilized metal affinity, and bioaffinity ligands.
Membranes and monoliths both offer dramatic advantages in binding capacity and throughput in addition to convenient handling. Monoliths compound those advantages with the high resolving capability necessary to support removal of contaminants with binding characteristics that are similar to those of a product. The low shear of monoliths can be expected to emerge as an important factor in the purification of live and attenuated viruses. Together, these benefits contribute to faster process development, higher product recoveries, more efficient process validation, shorter time to market, more economical manufacturing, and faster response to sudden demands for dramatically increased production — all of which promise to elevate the industry’s ability to fulfill the demands of this rapidly changing and evolving market.
REFERENCES
1.) Iberer, G, R Hahn, and A. Jungbauer. 1999. Monoliths As Stationary Phases for Separation of Biopolymers: The Fourth Generation of Chromatography Sorbents. LC-GC Int. 11:998-1005.
2.) Jungbauer, A. 2005. Chromatographic Media for Bioseparation. J. Chromatogr. A 1065:3-12.
3.) Švec, F, T Tennikova, and Z. Deyl. 2003.Monolithic Material: Preparation, Properties and Applications, Elsevier, Amsterdam.
4.) Afeyan, N. 1990. Flow Through Particles for the High Performance Liquid Chromatography Separation of Biomolecules. J. Chromatogr. 519:1-29.
5.) Podgornik, A, and A. Strancar. 2005. Convective Interaction Media (CIM): Short Layer Monolithic Chromatographic Stationary Phases. Biotechnol. Annu. Rev. 11:S281-S333.
6.) Hahn, R. 2002. Mass Transfer Properties of Monoliths. Sep. Sci. Technol. 37:1545-1565.
7.) Strancar, A. 2002. Short Monolithic Columns As Stationary Phases for Biochromatography. Adv. Biochem. Eng. Biotechnol. 76:49-85.
8.) Rodrigues, T. 2007. Purification of Retroviral Vectors for Clinical Application: Biological Implications and Technological Challenges. J. Biotechnol. 127:520-541.
9.) Lutsch, C, R Hjorth, and R. Pitiot. 2008.. Keynote Address: From State-of-the-Art to Future Challenges in Viruses and Viral Vaccines Purification.
10.) Gagnon, P. 2007.. Poster: A Comparison of Microparticulate, Membrane, and Monolithic Anion Exchangers for Polishing Applications in the Purification of IgG Monoclonal Antibodies.
11.) Giddings, J. 1965.Dynamics of Chromatography: Part I, Marcel Dekker, New York.
12.) Hearn, MT. 1982. High Performance Liquid Chromatography and Its Application in Protein Chemistry. Adv. Chromatogr. 20:1-64.
13.) Kirkland, J. 2000. Superficially Porous Silica Microspheres for Fast High-Performance Liquid Chromatography of Macromolecules. J. Chromatography A 890:3-13.
14.) Urthaler, J. 2005. Application of Monoliths for Plasmid DNA Purification Development and Transfer to Production. J. Chromatogr. A 1065:93-106.
15.) Etzel, M. 2008.. Charged Membranes and Monoliths in Chromatography.
16.) Qu, G. 2007. Separation of Adeno-Associated Virus Type 2 Empty Particles from Genome Containing Vectors By Anion-Exchange Column Chromatography. J. Virol. Met. 140:183-292.
17.) Gagnon, P. 1997. Avoiding Instrument-Associated Aberrations in Purification Scale-Up and Scale-Down. BioPharm 10:42-45.
18.) Hagel, L. Janson, JC and L 1989.Gel FiltrationProtein Purification: Principles, High Resolution Methods, and Applications, VCH Publishers, New York:63-106.
19.) Strancar, A. 1998. Convective Interaction Media: Polymer-Based Supports for Fast Separation of Biomolecules. LC-GC Int. 11:660-669.
20.) McGrath, M. 1998. Retrovirus Purification: Method That Conserves Envelope Glycoprotein and Maximizes Infectivity. J. Virol. 25:923-927.
21.) Slepushkin, V. 2003. Large-Scale Purification of a Lentiviral Vector By Size Exclusion or Mustang Q Ion Exchange Capsule. Bioprocessing J. 2:89-95.
22.) Jaalouk, D. 2003. Size Exclusion Chromatography Purification of High-Titer Vesicular Stomatitis Virus G Glycoprotein-Pseudotyped Retrovectors for Cell and Gene Therapy Applications. Hum. Gene Ther. 14:1139-1153.
23.) Jorio, H. 2007. High Yield Purification of Functional Baculovirus Vectors By Size Exclusion Chromatography. J. Virol. Met. 142:21-28.
24.) Peixoto, C. 2007. Downstream Processing of Triple Layered Rotavirus Like Particles. J. Biotechnol. 127:452-461.
25.) Morenweiser, R. 2005. Downstream Processing of Viral Vectors and Vaccines. Gene Ther. 12:S103-S110.
26.) Volkin, D. 2007. Size and Conformational Stability of the Hepatitis A Virus Used to Prepare VAQTA, a Highly Purified Inactivated Vaccine. J. Pharm. Sci. 86:666-677.
27.) Kalbfuss, B. 2007. Direct Capture of Influenza Virus from Cell Culture Supernatant with Sartobind Anion-Exchange Membrane Adsorbers. J. Membr. Sci. 299:252-260.
28.) Wu, C, K Soh, and S. Wang. 2007. Ion Exchange Membrane Chromatography Method for Rapid and Efficient Purification of Recombinant Baculovirus and Baculovirus gp64 Protein. Hum. Gene Ther. 18:665-672.
29.) Vicente, T. 2008. Anion-Exchange Membrane Chromatography for Purification of Rotavirus-Like Particles. J. Membr. Sci. 311:270-283.
30.) Spect, R. 2004. Densonucleosis Virus Purification By Ion Exchange Membranes. Biotechnol. Bioeng. 88:465-473.
31.) Maurer, E. 2008.. Influenza Vaccine Purification Platform.
32.) Smrekar, F. 2008. Purification and Concentration of Bacteriophage T4 Using Monolithic Chromatography Supports. J. Chromatogr. B 861:177-180.
You May Also Like