Appendix 1: Designing for Process Robustness
A rich cup of coffee is what comes to mind for many people when you mention the word robust. For biotechnologists it is often a comfortable term, generally referring to the overall strength or ruggedness of a manufacturing process. However, the origin of the robustness concept for manufacturing is found in the field of robust design, which has for decades been a rigorous discipline with its own metrics, algorithms, and mathematical tools. Lately it has experienced a renewed interest in further development of its formal approaches, mathematical modeling, and applications. Applications of robust design exist in many specialized disciplines from economics to engineering (including bioprocess engineering), with each emphasizing different elements of the concept.
Process Robustness
For bioprocessing professionals, an appropriate definition of process robustness is provided in ICH Q8: “ability of a process to tolerate variability of materials and changes of the process and equipment without negative impact on quality.” Although specific applications and means are not identified there, process robustness is an express goal in recent ICH guidelines and FDA guidances (1, 2). Thus, in the design of unit processing operations (UPOs), it should be considered as a means to maintain desired product or process characteristics by providing a degree of resiliency or insensitivity to variability or uncertainty in process inputs.
Figure 1:
NOVARTIS AG (WWW.NOVARTIS.COM)
Biological Systems and Robustness
Biological systems classically display both high complexity and robustness. From whole organisms to cellular functions, we find systems able to maintain functional performance in the face of environmental change.
For example, consider the lac operon, which permits host cells to seamlessly continue normal overall operation even as their energy source changes from glucose to lactose. This two-system regulatory network, though definitely increasing complexity, provides sensitivity and a means of stability for efficient operation in an altered environment.
Theorists describe such system robustness in terms of modular complexity, feedback control, or system interfaces supporting a dynamic interaction network. Others say these systems provide an inherently regulated redundancy and diversity — whereas we might say that “they’re ready for plan B.”
Complexity and Robustness
Some concepts in robust design are somewhat counterintuitive. For example, we often regard highly complex systems as fragile or even as operating on the edge of failure. This may be because highly complex man-made processes are seldom very robust. However, although this is often the case, it this does not necessarily need to be true. For example, feed-back control mechanisms using modular subsystems may greatly increase overall system complexity, but they can actually make a process much less fragile in many circumstances of variability.
Variability and Robustness
A first step in understanding variability (the potential or propensity to vary) in robust design is to consider different variabilities or sources of variability addressed in a process. For example, the ICH definition specifically mentions variability in materials. An immediate effect of materials variability can be variability in a manufacturing process. But the ultimate concern is consequent variability in the final product. Variation (existing differences in a parameter) in a process can arise from variability either internal or external to that process. From variation in process load and input variables to variation in material properties to noise in monitoring systems to process component failure, there are a many sources of variability in bioproduction.
Another issue is that the numerous terms used for variability have identical, very related, or slightly context-determined distinctions in meaning. People developing models of robustness often stress (and distinguish between) process input variation due to variability or uncertainty, people in electronic applications often refer to noise, and software engineers design to accommodate bugs or faults in software or interfaces. Whether termed input disturbance, change, failure, error, or common cause variability, for the most part we can consider all of these as sources of potentially disruptive perturbations to our systems.
“Quality by Design” and Robustness
The concept of robustness in bioprocessing can be observed in such diverse areas as biological systems, analytical procedures, equipment design, and control systems operation. By considering robustness as the ability of a system to tolerate defined sources of variability or uncertainty and maintain preestablished product quality, it’s clear that the ultimate objective is the same as that portrayed in the recent ICH QbD guideline (1). So there is great opportunity in a biomanufacturing process to ally robust design with QbD principals aimed at actually reducing such sources toward the very same end.
Robust Design
From a systems perspective, there are many approaches to dealing with variability that could lead to deterioration of a process (and its product) if left unaddressed. Robust design is a discipline occupied with improving product quality by minimizing the effects of process variabilities. However, it does not eliminate them. Rather, it acts to optimize the mean performance of a process and minimize variation by transferring each input variation to regulatory or control elements, thereby rendering the process less sensitive to disturbance.
One challenge for biotechnologists attempting to apply robust design in a particular UPO is that theory and derived models may present the fundamental principles and metrics of the field, but they can be quite abstract and mathematically challenging. Resources offering practical solutions (such as in the computer or mechanical engineering fields) can be so adapted to specific processes that it is difficult to extract any general guiding principles. Practical applications of robust design in bioprocessing thus requires identifying both the particular process step that needs to become more robust and what particular process variation is to be monitored for and manipulated by its control elements. For example, certain processes can be designed to be less sensitive to variability in raw materials or environmentally originated process disturbances. Cells in culture can be engineered to be more tolerant of variability in operating parameters, or a controller can be designed to better tolerate noise or error in sensor inputs.
Process engineers may improve the robustness of their processes by using an existing, formal, and structured design approach (e.g., a Taguchi-based method). Whereas some design problems are very responsive to prior analysis in models or simulations, others are simply too complex to be reduced to a set of equations, so they must be tackled using actual laboratory experimentation. Furthermore, robustness margins can be increased more informally, often relying on expert knowledge. Or they may be improved indirectly or accidentally as a consequence of some other optimization of the system.
Although the latter does actually occur at times, it is certainly not worth coun
ting on because it is not linked to process understanding. In fact, it can even result in a net reduction of such understanding. For example, increasing control capability with the specific intent of improving productivity could result not only in increased productivity, but a more robust process as well. However, the process is then desensitized to certain variabilities through an unknown mechanism, making it difficult to monitor or maintain.
It appears that we can expect an increased use of formal, prospective robust design methodologies in bioprocess development given
the success of formal robust design approaches in other industries;
new developments in statistics, modeling, and robust design theory itself
supporting technologies such as bioinformatics, chemometrics and systems biology.
This supplement reviews some ways increased process understanding can reduce process or product variation in manufacturing and thus increase product quality. Improved process understanding can identify sources of previously unknown variability, assist in reducing or even eliminating such variabilities, and be used to help to make a process more tolerant to such variability. QbD and PAT approaches highlight the need for process understanding as a prerequisite to reducing process variability, and it is clear that robust process design is an important tool in accomplishing that goal.
Appendix 2: Process CharacterizationIn Search of Platform Technologies
Biopharmaceutical manufacturing processes using in vitro cellular expression systems to produce therapeutic proteins depend on the complex and dynamic chemistries of living cells (1,2,3). These systems posses significantly higher intrinsic variability than most synthetic processes, making the development and characterization of reliable and robust manufacturing processes an arduous task. Process characterization studies need to describe the performance of individual process steps, including defining operational parameters (critical, key, and nonkey) and setting acceptable ranges (limits) and acceptance criteria for validation protocols. For example, the unique operation of an anion-exchange chromatography purification step requires batch-binding studies to establish the fundamentals of the separation mechanism involved.
Although each biopharmaceutical is unique, and no two approaches to process development and optimization are exactly alike, they often have many common or closely related process steps unit processing operations (UPOs). This allows standardization of individual process steps in a system known as a platform. Platforms consist of well-characterized process steps and promise the process versatility to support manufacturing of a broad, molecularly related class of biopharmaceuticals such as monoclonal antibodies (MAbs). Such products must be similar and well-characterized enough to allow for a core of process steps and operating ranges (design space), but they can reflect significant diversity in a number of properties from antigen and epitope specificity to protein molecular weight, isoform, and isoelectric point. Their general similarity is what allows for a consistent platform of UPOs to be used, but their diversity requires different operating windows (control spaces) to be set for each process step for each product.
Although public access to the details of biopharmaceutical manufacturing processes are often lacking, what is published reveals a pattern that allows description in a rather abstracted overview. Figure 1 shows a generalized manufacturing process highlighting individual UPOs. The product accumulates either intracellularly (e.g., bacterial culture–based processes in which recombinant proteins make up inclusion bodies) or is secreted (e.g., mammalian cell culture processes). The figure also shows viral inactivation and removal steps that are unique in eukaryotic-based manufacturing processes (4). This generalized process flow diagram (PFD) starts when a vial of the working cell bank (5) is retrieved and a culture is established and expanded in a progressive schedule of increasing volume. Such cultures are routinely monitored for cell density, viability, and productivity, with critical operating parameters (e.g., pH, DO, impeller speed, pressure, and gas flow) controlled where required. After production, clarification and purification steps remove process- and product-related impurities and contaminants, while protecting product quality. The concluding process steps, sometimes performed by specialized manufacturer’s subsidiaries or contract manufacturers, include compounding or formulation of the active pharmaceutical ingredient (API) with excipients, followed by sterile filtration, filling, and sometimes lyophilization to produce a finished product. This abbreviated model illustrates the complexity of modern recombinant protein manufacturing processes, their typically applied UPOs at commercial scale, and the scope of the underlying process characterization efforts.
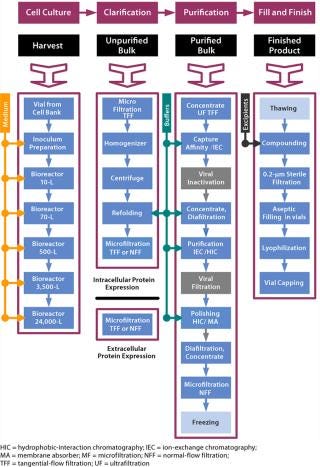
Figure 1: ()
Established biopharmaceutical producers and first-product biotech companies alike spend a considerable amount of resources both in development of their products and in design and control (as part of process characterization) of their manufacturing processes. Unlike first-product sponsors, however, established companies with a product portfolio on the market have acquired manufacturing experience and a proven infrastructure. That gives them an advantage and a desire to standardize UPOs to establish platforms. Nevertheless, this has remained an elusive goal for all and has been met so far with only limited success in, for example, MAb production processes.
Appendix 3: Product CharacterizationRelevant Product Attributes
Biomanufacturers put considerable up-front effort into characterization of their products. These efforts follow a distinct product-specific approach, which directly results from the complex molecular characteristics of individual proteins (Figure 2). Characterization of any protein biologic includes determination of its purity and physiochemical, biological, and immunochemical properties. Although regulatory guidelines have been provided, and the establishment of specific characteristics such as potency is required, many particular critical quality attributes (CQAs) and analytical procedures for their assessment are not strictly defined. In fact, manufacturer-based determination of the most relevant product-specific quality attributes and analytical technologies is specifically encouraged.
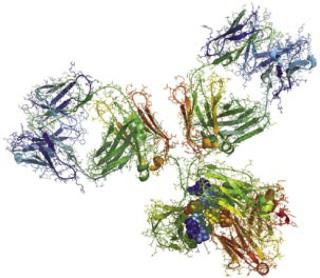
Figure 2: ()
Analytical Technology
For those who have recently entered the field of protein characterization, the rudimentary level of understanding in the structure of complex proteins existing in academia in the 1980s and even industrially in the early 1990s seems surprising. In less than two decades, analytical capabilities and structural knowledge have excelled through dramatic increases in the power, availability, and extent of the current arsenal of biomolecular methods and instrumentation. This has expanded the ability of scientists to detect finer levels of molecular diversity, but it has also increased industry and regulatory pressure for better-characterized products.
Instrumentation and analytical methods have evolved to enable more complete characterization of biopharmaceutical products than ever before, giving rise to the term well-characterized biotechnology products. That was originally conceived in 1995 by the FDA to describe, for example, monoclonal antibody products for in vivo use and therapeutic recombinant DNA-derived products. Determining the complete and absolute peptide sequence (primary structure) of proteins has become routine. Despite the enormous progress in bioanalytics, however, full characterization (higher-order structures) and mapping of posttranslational modifications (PTMs) of large, highly processed and multimeric proteins is not only time consuming, but difficult and sometimes unattainable.
Product Profile
Often the desired product is actually a mixture including anticipated posttranslationally modified forms (molecular variants) such as glycoforms. Such products are more accurately described by means of product profiles. Product-related variants that have properties comparable to those of the target protein in terms of activity, efficacy and safety are referred to as product-related substances (Figure 3). Hence, identity tests are highly specific to each particular product and based on unique and significant aspects of molecular structure or other specific properties such as function (Table 1). Typically, more than one assay providing even a significant characteristic is required to establish identity.
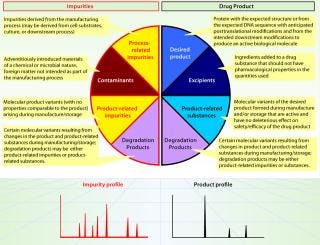
Figure 3: ()
Table 1: Protein characterization scope for structural and property identification and list of example bioanalytical technologies for their assessment.
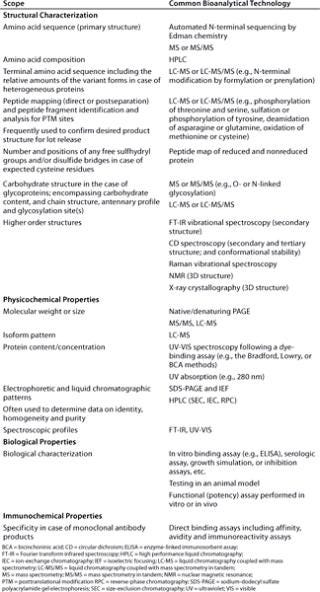
Table 1: Protein characterization scope for structural and property identification and list of example bioanalytical technologies for their assessment. ()
Impurity Profile
One of the most critical aspects of product characterization is the purity assessment generally described by an impurity profile. Adding to the challenge is the fact that the absolute and relative purity of a desired product is often difficult to determine. To this end, a combination of specific analytical assays is usually used to focus on separation of the desired product and product-related substances from their degradation products and other product- or process-related impurities (Figure 3, Table 2). Impurities can be of known structure, partially characterized, or even remain unidentified. Contaminants do not originate from manufacturing/storage activities, but rather consist of materials adventitiously introduced to a process, so they are captured in a separate category.
Table 2: Principal categories and sources of impurities (including degradation products) formed during biopharmaceutical manufacturing and/or storage, with potential contaminants and a list of common bioanalytical technologies for their assessment.
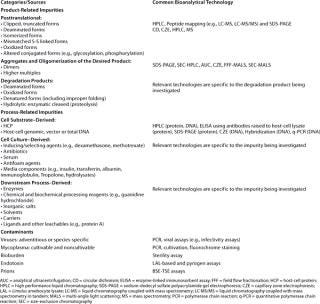
Table 2: Principal categories and sources of impurities (including degradation products) formed during biopharmaceutical manufacturing and/or storage, with potential contaminants and a list of common bioanalytical technologies for their assessment. ()
Setting Specifications
Biopharmaceutical product quality specifications are typically described in terms of often complex product and impurity profiles. These are often codetermined and always linked to a particular manufacturing process. Acceptance criteria — stipulated as an integral part of the specifications — are usually established and justified time-progressively (e.g., following a life-cycle approach) based on relevant data becoming available from process and product development, manufacturing lots of preclinical and clinical trial materials, and stability studies (1). This establishment and justification process is mostly heuristic and far from trivial because it is based on often initial limited product knowledge and process understanding, which can lead to product attribute acceptance limits that are too broad, unnecessarily tight, or completely irrelevant to clinical performance. Even worse, some critically important attributes may be missed during development. Ultimately, the level of process understanding that can be attained depends on the available analytics, time-line, and budget allotted to achieve the goals of a development program.
Product Comparability
Advances in product characterization and the ability to assess well-characterized biotechnology products by analytical assays have allowed regulatory authorities to develop scientific policies on product comparability (2). These have become a cornerstone of today’s product development programs. Protein characterization begins early in development to ensure that supporting data
are available to demonstrate the comparability of material produced throughout development and an evolving manufacturing processes. This also requires development of properly qualified in-house reference standards with known characteristics, potency, and specificity. Compendial or other reference standards for most new biotherapeutics are unavailable.
Process Proteomics
It is tempting to think of transferring the analytical technologies used for characterization directly as sensors for in-process monitoring and even in-process control for on-line and real-time settings (process proteomics). Unfortunately this is not likely to become common practice among bioprocessors in the immediate future because of method and systems complexities and constraints (e.g., toward elucidation of higher-order protein structures). Nevertheless, especially thanks to significant advances in analytical technologies and bioinformatics, this pursuit holds merit to further improve the manufacture of biopharmaceuticals. In lieu of process proteomics, information from characterization studies is used for development of suitable and validatable quality assays (3) assessing identity, purity, and potency (biological activity), which are critical in support of product manufacturing.
APPENDIX 4: INDUSTRY RESOURCES AT A GLANCE
Today, a comprehensive support network is available through user communities across the biopharmaceutical industry. These include many professional societies and associations.
American Association of Pharmaceutical Scientists (AAPS) www.aapspharmaceutica.com: Relevant here are activities of the analysis and pharmaceutical quality (APQ), biotechnology (BIOTEC), pharmaceutics and drug delivery (PDD), and pharmaceutical technologies (PT) sections, as well as particular activities of the focus groups on PAT; process development (PD); and manufacturing, engineering, and quality (MEQ).
American Society Testing Materials (ASTM) www.astm.org: Relevant here are activities of the technical committees E48 on biotechnology and (most notably) E55 on the manufacture of pharmaceutical products.
California Separations Science Society (CASSS, www.casss.org) focuses on the science of separation and biomolecular methods, especially their application to biopharmaceuticals and PAT. The Well Characterized Biotechnology Pharmaceutical (WCBP) meetings and WCBP CMC strategy forums are of particular interest.
International Forum on Process Analysis and Control (IFPAC, www.ifpac.com) is dedicated to PAT applications and implementation approaches.
Instrument Society of America (ISA, www.isa.org) is a truly international society dedicated to system control and automation standards. The ISA88 and ISA 95 committees have published a series of standards respectively on batch control in industrial automation systems and interfaces between enterprise activities and control activities.
International Society for Pharmaceutical Engineering (ISPE, www.ispe.org) is a large and very active society focusing on broad needs of pharmaceutical manufacturing professionals. Of particular interest here are the plentiful pharmaceutical engineering guides, good practice guides, and GAMP publications as well as numerous meetings addressing QbD and related topics. Recently, ISPE launched a multiyear global industry-led initiative referred to as the Product Quality Lifecycle Implementation (PQLI), which is aimed at facilitating implementation of a recent ICH guidance.
Parenteral Drug Association (PDA, www.pda.org) is a leading global provider of science, technology, and regulatory information and education for the pharmaceutical and biopharmaceutical community. Of particular interest are technical reports that provide recommendations and best practices on many pharmaceutical and biopharmaceutical topics where little or no other guidance exists.
These organizations provide active forums and information regarding the implementation of contemporary industry themes including QbD, QRM, and PAT. Several focus on the current state and progress of technologies and their adaptation to biopharmaceutical processes, including sampling and process analyzers, chemometrics, advanced control implementations, and modern quality and risk approaches.
REFERENCES
1.) 1985.Points to Consider in the Production and Testing of new Drugs and Biological Produced by Recombinant DNA Technology, US Food and Drug Administration, Rockville.
2.) ICH Harmonized Tripartite Guideline 2005..
3.) 1985.Points to Consider in the Production and Testing of new Drugs and Biological Produced by Recombinant DNA Technology, US Food and Drug Administration, Rockville.
4.) ICH Harmonized Tripartite Guideline 2005..
5.) 1997.Points to Consider in the Manufacture and Testing of Monoclonal Antibody Products for Human Use, US Food and Drug Administration, Rockville.
6.) ICH Harmonized Tripartite Guideline 1999..
7.) ICH Harmonized Tripartite Guideline 1997..
8.) ICH Harmonized Tripartite Guideline 1995..
9.) ICH Harmonized Tripartite Guideline 2004..
10.) ICH Harmonized Tripartite Guideline 2005..
You May Also Like