Bridging the Gap from Reusable to Single-Use Manufacturing with Stirred, Single-Use Bioreactors
During the past few years, use of disposable bioreactors in development and manufacturing processes has become widely accepted. Particularly, low–oxygen-demanding cell types such as human and insect cells have proven to be perfectly suitable for cultivation in single-use bag chambers. These bioreactors have significant advantages over their reusable counterparts (1). They transform a single-purpose process using stainless steel reactors into a multipurpose facility in which switching from one application to another is both easy and cost effective.
Reusable stainless steel bioreactors have fixed vessel configurations with predefined port assemblies, whereas single-use bioreactors use presterilized plastic cultivation chambers that get discarded after use. That makes adapting such cultivation vessels to specific applications highly convenient. Because single-use bags are presterilized by their suppliers, sterilization/cleaning is not required. That eliminates space-consuming and expensive clean-in-place (CIP) and steam-in-place (SIP) installations while reducing production turnaround times. With associated efforts for validation reduced, this ultimately shortens time to market and provides a faster return on investment.
Challenges to Overcome
Despite the advantages of single-use bioreactors, use of this technology is mainly restricted to development and seed-train applications. Wave-motion and stirred-motion bioreactors have become the standard on the market for single-use cell cultivation. The growing number of disposable-bioreactor suppliers has led to rapid increase in maturity for these technologies, providing a wide range of systems that can offer high cell densities and product titers. Until now, however, some difficulties remain to be overcome before single-use bioreactors can make a successful transfer to commercial manufacturing processes.
Reusable bioreactors are clearly characterized in terms of their performance, optimization steps, and design. They have been the “gold standard” for many decades, so they are supported by a large amount of knowledge and experience on process control at the operator side. Use of disposable bioreactors based on a similar design therefore builds a certain safety and robustness into your process.
Scalability is essential for manufacturing processes. Developers of industrial cell culture processes for production of recombinant proteins seek high efficiency, reproducibility, and predictability. Usually the time allotted for process development is short, during which culture conditions and scale-up protocols must be defined to maximize cell productivity and yield while minimizing process scope and overall cost. Having identically designed bioreactors throughout a complete manufacturing process facilitates up-scaling significantly.
Single-use bioreactors are currently limited to ≤2,000 L in scale. If a manufacturing process requires larger volumes, stainless-steel bioreactors or multiple disposables will be necessary. This explains the popularity of hybrid solutions, in which disposable bioreactors are used up to the 200– 1,000-L scale. In the next up-scaling step, culture broth from those cultivations is then transferred into a large-scale stainless steel bioreactor. So there is a clear need for single-use bioreactors with design and set-up that are comparable to the reusable alternative. The more unfamiliar a technology, the more questions both users and regulators will have.
Although multiple stirred, single-use bioreactors are widely available for implementation into cell culture processes, most are limited when it comes to this need for comparability. Some examples include eccentric stirrer motions, nonvertically installed stirrers, and deviations from standard impeller and sparger types.
Stirred-tank reactors (STRs) are the most commonly used bioreactors to cultivate suspension cells, mainly because of broad experience obtained in microbial fermentation using the same type of culture vessels. General design criteria have been derived from those microbial cultures and modified to meet the requirements of mammalian cells. Specifically, the shear sensitivity of animal cells requires consideration in the design of impellers, aspect ratio, and oxygenation. Scale-up principles for these reactors are generally better characterized than those of other bioreactor types.
Recently, Sartorius Stedim Biotech developed an alternative to existing stirred single-use bioreactors (Photo 1). Currently available as a single or twin version with a maximum working volume of 200 L, this system is soon to come in 50-L, 500-L, and 1,000-L sizes. In a twin system, a single controller simultaneously controls two independent cultivations, which reduces investment costs and necessary floor space. Our focus in developing this bioreactor (trade-named BIOSTAT CultiBag STR) was to improve comparability of single-use and classical processes and thus facilitate integration of single-use bioreactor technologies into modern cultivation processes. To assure this comparability, several critical design criteria were taken into account.
Photo 1:
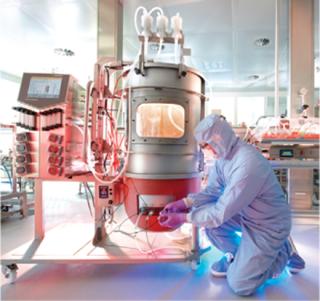
Photo 1: ()
Design Criteria
VESSEL DESIGN: Typical aspect ratios (height/diameter) for stirred-tank reactors vary between 1:1 and 3:1 depending on their application. At low aspect ratios, gas transfer through the vessel headspace is generally good because of a relatively high surface-to-volume ratio. But higher aspect ratios offer certain advantages when direct sparging is used for oxygenation. Better dispersion and longer residence times for gas bubbles in the culture liquid improve gas transfer rates.
A typical vessel for cell culture applications has a height/diameter ratio of 2:1 and maximum filling volume of 70–80%. A harvesting port is installed at the lowest point of the vessel to keep dead volume to an absolute minimum. In single-use bioreactors, a bag holder is needed to assure integrity of bag-design characteristics during cultivation. The disposable bag needs to fit perfectly to its holding device to prevent irregularities against a classical cultivation vessel design caused by bad unfolding during setup. To guarantee comparability with reusable processes, all these requirements have been integrated into the design of the system (Photo 2).
Mixing Mechanism: Agitation in stirred-tank reactors is intended to provide homogeneous suspension of cells and prevent chemical (nutrients and waste products), physical (pH, oxygen, and carbon dioxide), and thermal gradients inside the vessel. Large impellers with axial fluid flow characteristics (e.g., segmented impellers) are typically used to minimize shear rates. As reactor size increases — especially with increasing height — it becomes necessary to install multiple impellers to prevent fluid compartmentalization. With the same power input, multiple-impeller systems require lower impeller speeds for the same results, which lowers the intensity of local energy dissipation (2).
Photo 2:
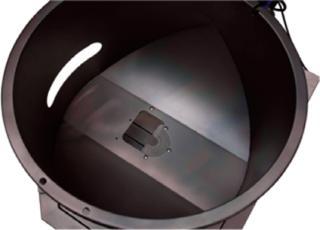
Photo 2: ()
Comparative studies using different impeller types with an axial flow pattern in a shear-sensitive inorganic test system revealed that the lowest shear forces (as determined by floccular disruption) were obtained with a three-blade segment impeller (Photo 3) (3). Such an impeller allows for efficient mixing with low shear stress. This makes our new bioreactor suitable not only for suspension cell lines, but also for microcarrier cultures.
Photo 3:
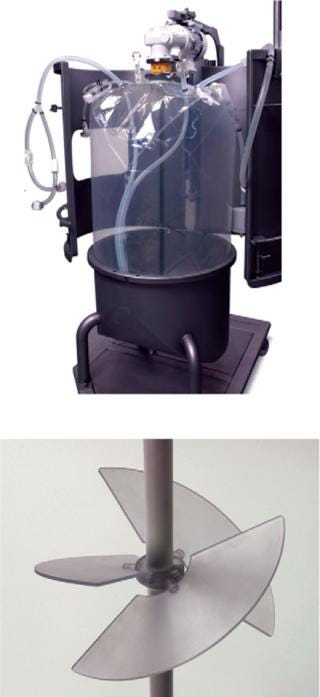
Photo 3: ()
The Rushton impeller is another type that can be integrated into a CultiBag STR bioreactor. It is the most common flat-bladed or disk-turbine impeller such as a six-blade disk impellers (Photo 4). The blades are flat and set vertically along an agitation shaft, which produces a unidirectional radial flow. Rushton and Rushton-type impellers are often used in culture of cell lines not considered to be shear sensitive, including bacteria, yeasts, and some other fungi. However, a Rushton impeller also can be used as the bottom impeller to disperse bubbles in a bioreactor. Combining a radial-flow six-blade disk impeller with an axial-flow three-blade segment impeller can provide beneficial flow distribution for some applications (4).
Photo 4:
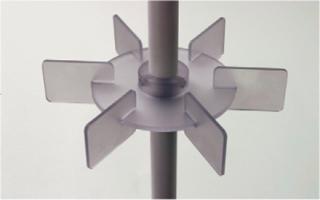
Photo 4: ()
A CultiBag STR stirrer is installed from the top in a vertical position at the center of the single-use bag. It is completely part of the bag and thus comes preinstalled from the supplier, which reduces the risk of bag damage during stirring because it uses no mechanical seals. This also lowers the required height of a single-use facility because no stirrer axe needs to be installed from the top after installation of the disposable bag (which is the case with some other stirred, single-use bioreactors).
In a CultiBag STR 200 bioreactor, the ratio diameter of impeller to bag is 0.38, which correlates to the classical ratio of 0.33–0.5 (5). The angle of the blades (30°), the positioning and number of impellers, and their diameter provide for efficient mixing even at low speeds (Table 1). Calculation of the corresponding Reynolds number — which is firmly above 60,000 — indicates a turbulent flow and therefore efficient mixing.
Table 1: Experimental results for mixing times in a BIOSTAT CultiBag STR 200 bioreactor using the concentration method and two three-blade segment impellers
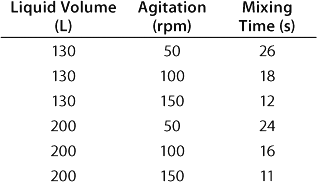
Table 1:
Table: Experimental results for mixing times in a BIOSTAT CultiBag STR 200 using the concentration method and two six-blade disk impellers
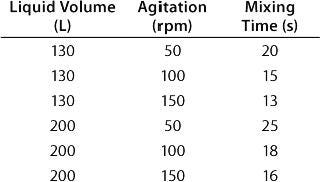
Table: Experimental results for mixing times in a BIOSTAT CultiBag STR 200 using the concentration method and two six-blade disk impellers ()
Mixing characteristics for the two impeller configurations (a six-blade disk impeller with three-blade segment impeller and two three-blade segment impellers) were determined using the iodinethiosulfate-decolorization and concentration methods. Mixing time determined by the concentration method was investigated by measuring conductivity in distilled water with addition of potassium phosphate buffer. For the two impeller configurations, both methods showed similar mixing times, so both impeller configurations were shown to offer efficient mixing (Table 2).
Table 2: Calculated results for tip speed in a BIOSTAT CultiBag STR 200 bioreactor with impeller diameter of 225 mm
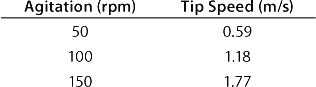
Table 2:
Aeration: Minimization of mechanical forces is not the only design criterion for oxygenation systems. Sufficient oxygen (O2) transfer and carbon dioxide (CO2) removal are also important in selection of a bioreactor system.
Oxygen is a key nutrient in cellular metabolism. Even though the oxygen demands of animal cell cultures are several orders of magnitude lower than those of microbial cultures, oxygenation is often the primary challenge in cell-culture scale-up. The importance of aeration increases with bioreactor volume and cell concentration. Whereas low oxygen concentration affects cell growth and yield, excessive oxygen tensions can also be toxic to cells (4).
The classical and commonly used aeration method is direct sparging, bubbling gas directly into the culture medium. Proper sparger selection is important for increasing aeration and minimizing cell damage and foaming. Air or oxygen is sparged into animal cell cultures with ring spargers (drilled holes >0.5 mm diameter) similarly designed to those used in microbial fermentors or with microspargers made of sintered materials (with pore sizes of 10–100 µm). Microspargers provide significantly higher gas-medium interfaces and thereby higher O2 mass-transfer coefficients with the same gas flow rate, which effectively reduces the overall superficial gas flow rate (2). Both types of spargers (Photo 5) are standard in CultiBag STR systems, in which they are installed below the stirrer device for efficient gas transfer.
Photo 5:
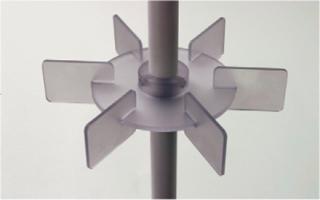
Photo 4: ()
Despite their mass transfer efficiency, very small bubbles (www.sigmaaldrich.com) are typically added to cell culture media. But damage can still occur to those in the vicinity of bubble ruptures. Larger bubbles have lower maximum energy dissipation rates associated with rupture, which is consistent with literature observations that larger bubbles are less damaging to cell cultures (6).
Addition of air or pure oxygen to a system can, and usually does, remove CO2 from that system. Because both CO2 accumulation and excessive removal should be avoided, increases in O2 transfer rate (OTR) must be balanced for the negative effect it has on removing CO2 from the system (4). With larger bubbles, higher volumetric gas flows are required to control dissolved oxygen, and CO2 is more effectively removed. Smaller bubbles also lead to generation of more stable foam layers, requiring higher concentrations of antifoam agents. For all these reasons, larger bubbles (~5 mm) are preferred in industrial cell culture applications (6).
Even if other aeration methods are used, flushing air through the headspace compartment of a bioreactor provides an additional means to increase the overall OTR and additionally remove CO2. Maintaining a balance between O2 addition and CO2 removal leads to more complexity with respect to gas exchange.
CONTROL LOOPS: When more oxygen is demanded by cells in culture, one possibility is to increase the gas flow going to the sparger. Too high a gas flow will lead to bubble coalescence, however, and decrease the gas–liquid interphase (4). For this reason the BIOSTAT CultiBag STR system is equipped with a completely flexible, automated gassing system that can change the composition of the incoming gas. A mixture of air, O2, nitrogen (N2), and CO2 is brought into the cell culture depending on feedback coming from dissolved oxygen (DO) and pH sensors.
With a clear need for multipurpose facilities in the biopharmaceutical industry, this gassing system allows operators to quickly and easily change gassing directions. Two gassing lines go into the single-use bag, one for the headspace and one for sparging, but as many as six independently controlled gassing lines are behind this strategy (Figure 1). Operators can direct those lines as they prefer. An overlay line brings the standard-configuration two gassing lines (air and CO2) into the headspace. A sparging line brings up to four different lines to the sparger (air, O2, N2, and CO2). Alternatively, an operator can redirect manually O2 and N2 from the sparger to the overlay, thus bringing four gasses into it. This flexibility gives operators complete control of their cultivations and enables them to change their processes without problems either during cultivation or in changing from one application to another.
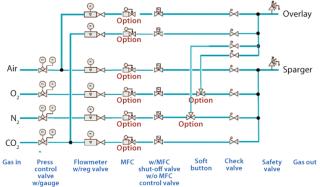
Figure 1: ()
Increasing stirrer speed represents another control loop that can lead to a higher OTR. It is limited by increasing shear stress. All these alternatives can bring significant benefits to a cultivation process. The BIOSTAT CultiBag STR control software is therefore equipped with a multistage cascade system for maintaining the level of dissolved oxygen. Thus, an operator can bring all different control possibilities into one cascade, and the control software decides automatically which is most suitable at a given stage. At any time, the operator can change priorities, proportional–integral–derivative (PID) parameters, limits, and all other relevant factors without trouble. For central control and data storage of multiple bioreactors, several BIOSTAT CultiBag STR systems can be connected together with other types of bioreactors to an advanced BioPAT MFCS/Win supervisory control and data acquisition (SCADA) software system.
Scale-Up
Because scaling up/down is not an exact science, it is often based on a combination of multiple cultivation characteristics. Given the various challenges involved, knowledge and experience are nearly as important as the scale-up parameters (4). Being designed as a classical bioreactor, the BIOSTAT CultiBag STR system allows its operator to use his or her experience to facilitate up-scaling.
Selection of appropriate mass-transfer conditions is important to achieving consistent cell culture and fermentation performance across sites and scales. In general, tank geometry and hardware (impellers, sparger, and so on) are not subject to change during scale-up. So the focus is on defining appropriate agitation and sparge-gassing conditions to achieve consistent performance (6).
Scale-up predictors using empirical calculations should be applied to assess large-scale bioreactor capability regarding gas flows and agitation speeds in relation to constant power input per unit volume (P/V), tip speeds, superficial gas velocities, and predicted KLa values. However, it is not possible to hold more than one of these parameters constant at the same time during scale-up (4). So it is important to determine the critical parameter(s) in a given bioreactor and use that in an adequate scale-up strategy.
Shear Rate and Tip Speed: Another agitation-related parameter often evaluated, especially for cell culture applications, is shear rate. Because mammalian cells lack a cell wall, they are more susceptible to shear damage than are microbial cells. Impeller tip speeds can be correlated to maximum impeller shear rates, so constant tip speed has been suggested as a scaling criterion for mammalian cell culture agitation. As a guideline, this parameter should lie between 1 and 2 m s-1 during scale-up (2). Calculations have shown that the new stirred, single-use bioreactor also fulfils this demand (Table 2). However, multiple investigators have found that impeller shear rates commonly used for cell culture applications are orders of magnitude below those required to cause cell damage (7). So we do not recommend using tip speed as a primary agitation scaling parameter.
Power input per volume or the mean specific energy dissipation rate is a parameter more commonly used for scaling agitation across sites or scales. The power input per unit volume in animal cell cultures is typically much lower than that in microbial cultures because of the assumed higher fragility of mammalian and insect cells and their significantly lower oxygen uptake rates. Quantitatively, this agitation intensity difference is 100-fold: a global average of 10 W/m3 (1–50 W/m3) in animal cell culture vessels compared with 1,000 W/m3 in microbial fermentation vessels (6).
The power input per volume was calculated by measuring torque in a CultiBag STR 200 bioreactor (8) with a liquid volume of 200 L distilled water and an agitation rate of 100 rpm. A power input per volume of 17.5 W/m3 was determined using two two-blade segment impellers. With a six-blade disk impeller and three-blade segment impeller configuration, a power input per volume of 32.2 W/m3 was achieved.
Superficial Gas Velocity: The overall gas flow rate should be scaled separately with respect to minimum and maximum sparge gas flow whereas the combined minimum sparge rate was scaled on constant vvm and the combined maximum sparge rate was scaled on constant superficial velocity (vs) to minimize foam generation (9). For animal cell cultivation, specific aeration rates should be lower than 0.1 vvm (10). For this rate, we calculated a maximum superficial gas velocity of 0.0012 m/s for the CultiBag STR 200 system.
Volumetric Mass-Transfer Coefficient (KLa Value): Animal cell cultures require oxygen for production of energy and formation of product from organic carbon sources. Oxygen can be a limiting factor for animal cell cultivations because of its low solubility in water and high rate of consumption by cells. The specific oxygen uptake (consumption) rate is cell-line dependent. For example, at 37 °C it can be 0.31 pmol/cell/h for Chinese hamster ovary (CHO) cells, 0.30 pmol/cell/h for baby hamster kidney (BHK) cells, and 0.22 pmol/cell/h for hybridoma cells (11). Specific values will also depend on a number of factors such as cell growth rate.
Volumetric mass-transfer coefficients were measured in a CultiBag STR 200 bioreactor for both impeller configurations using the gassing-out method (12). The experiments were conducted using water at 37 °C and an agitation rate of 150 rpm, with gas flow rates of 3 L/min for the ringsparger and 20 L/min for the headspace.
The determined KLa values were ~4.5 L/h for the two three-blade segment impellers and 7.4–10.5 L/h for the six-blade disk impeller and three-blade segment impeller configuration (Table 3). Those KLa values are similar to or higher than those published for most cell culture bioreactors (13). With only headspace aeration in a CultiBag STR 200 bioreactor, KLa values of ~1 L/h were determined for both impeller configurations, so sparger aeration is shown to be more efficient than the headspace aeration alone.
Table 3: Experimental results for KLa value in tapwater at 150 rpm and 37 °C with a ring sparger gas flow rate of 3 L/min in a BIOSTAT CultiBag STR 200 bioreactor
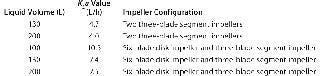
Table 3: Experimental results for KLa value in tapwater at 150 rpm and 37 °C with a ring sparger gas flow rate of 3 L/min in a BIOSTAT CultiBag STR 200 bioreactor ()
The volumetric mass-transfer coefficient is primarily a function of power input per volume and the superficial gas velocity. Thus, higher gas flow rates will increase KLa values. Tests are ongoing. Another option to increase the KLa value would be aeration with pure oxygen.
Bridging the Gap
The new stirred, single-use bioreactor described herein can bring significant improvements to an animal cell cultivation process. Like other single-use bioreactors, it makes cell culture operations more flexible, cheaper, and less time consuming. And thanks to its correlation with the classical design of reusable bioreactors, this can remove existing challenges and therefore represent an important step toward complete maturity of single-use technologies. So the system can assist in creating a modern, flexible, multipurpose facility for biopharmaceutical production.
REFERENCES
1.) Eibl, D, and R. Eibl Eibl, R. 2008.Bioreactors for Mammalian Cells: General OverviewCell and Tissue Reaction Engineering, Springer, Germany.
2.) Fenge, C, and E. Lüllau Ozturk, SS and W-S. 2006.Cell Culture BioreactorsCell Culture Technology for Pharmaceutical And Cell-Based Therapies, CRC Taylor & Francis Group, Boca Raton.
3.) Fenge, C. 1993. Agitation, Aeration and Perfusion Modules for Cell Culture Bioreactors. Cytotechnol. 11:233-244.
4.) Ma, N, M Mollet, and JJ Chalmers Ozturk, SS and W-S. 2006.Aeration, Mixing and Hydrodynamics in BioreactorsCell Culture Technology for Pharmaceutical And Cell-Based Therapies, CRC Taylor & Francis Group, Boca Raton.
5.) Marks, D. 2003. Equipment Design Considerations for Large Scale Cell Culture. Cytotechnol. 42:21-33(13).
6.) Rathore, AS. 2008. Modeling of Biopharmaceutical Processes — Part 1: Microbial and Mammalian Unit Operations. BioPharm Int. 21:56-65.
7.) Ma, N, KW Koelling, and JJ. Chalmers. 2002. Fabrication and Use of a Transient Contractional Flow Device to Quantify the Sensitivity of Mammalian and Insect Cells to Hydrodynamic Forces. Biotechnol. Bioeng. 80:428-437.
8.) Holland, IA, and FS. Chapman. 1966.Liquid Mixing and Processing in Stirred Tanks, New York, NY, Reinhold.
9.) Seamans, S. 2008. Cell Cultivation Process Transfer and Scale-Up in Support of Production of Early Clinical Supplies of an Anti IGF-1R Antibody, Part 2. BioProcess Int. 6:34-42.
10.) Czermak, Eibl, R 2008.Special Engineering AspectsCell and Tissue Reaction Engineering, Springer, Berlin.
11.) Jorjani, P, and SS. Ozturk. 1999. Effects of Cell Density and Temperature on Oxygen Consumption Rate for Different Mammalian Cell Lines. Biotechnol. Bioeng. 64:349-356.
12.) Wise, WS. 1951. The Measurement of the Aeration of Culture Media. J. Gen. Micro. 5:167-177.
13.) Nehring, D. 2004. Experimental Study of a Ceramic Mircosparging Aeration System in a Pilot Scale Animal Cell Culture. Biotechnol. Prog. 20:1710-1717.
You May Also Like