A Prescriptive Approach to Management of Solid Waste from Single-Use Systems
In biopharmaceutical manufacturing, the disposal of solid waste from single-use systems is becoming an increasingly important issue. The new focus is driven by several major factors including a broadening range of disposable technologies enabling, in some cases, the installation of completely disposable multistage systems; improved scalability of single-use components offering production capacities to thousands of liters; and the environmental impact of waste disposal. The latter concern includes not only regulatory and cost constraints, but also the need for users to implement a responsible approach for environmental sustainability.
PRODUCT FOCUS: ALL BIOLOGICS
PROCESS FOCUS: MANUFACTURING
WHO SHOULD READ: PROCESS DEVELOPMENT, FACILITIES MANAGEMENT, AND MANUFACTURING
KEYWORDS: DISPOSABLES, DISPOSAL, ENVIRONMENTAL IMPACT, SINGLE-USE TECHNOLOGY
LEVEL: BASIC
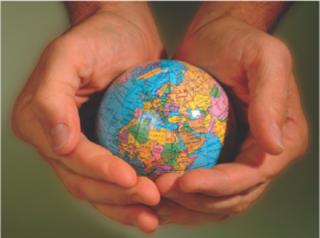
Figure 1: Environmental impact can and should be part of the single-use equation.
All those factors must be balanced against the potential benefits of single-use systems over those of traditional stainless steel processes (1,2,3,4). For example, disposables generate more solid waste but consume much lower quantities of water, chemicals, and energy to use. Sinclair et al. compared a model stainless steel system with a single-use system on a scale of 3 × 2,000 L (5). They calculated that the total water use (both water for injection, WFI, and purified water, PW) for a disposable system was nearly eight times lower (Figure 1), and the use of cleaning chemicals was more than 20 times lower. Similar calculations for energy consumption of model systems showed that the combined value for sterilization, cleaning, and materials was ∼50% lower for the single-use system (Figure 2). These benefits, which often provide a net cost savings, can also translate to a more favorable environmental impact, which may be further enhanced by a structured approach to waste management of single-use components.
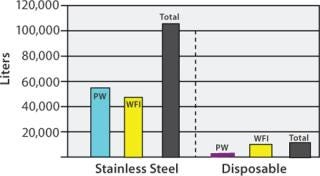
Figure 1: Water use for model systems (5)
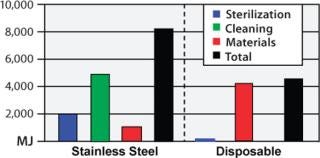
Figure 2: Energy consumption for model systems (6)
Model Disposable System
The model system illustrated in Figure 3 represents the main components of a process typically found in biotechnology manufacturing for products such as monoclonal antibodies (MAbs) at 2,000-L scale. To simplify the assessments described below, this model does not include every component in a process. For example, a typical process may contain as many as 30–90 bags and biocontainers used for buffers, media, product, flush liquids, and product samples.
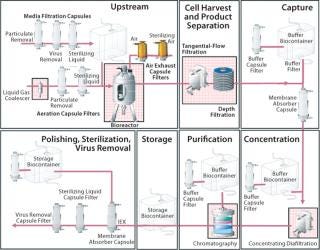
Figure 3: Model disposable bioprocess system
Note that the model contains not only single-use, but also some multiuse, cleanable unit operations, which are indicated by a red background. Such hybrid systems are often found in large, multistage processes in which some traditional stainless steel components have been retained due to a lack of suitable single-use alternatives, limitations on scalability, or substantial existing investment in stainless steel equipment.
Although the terms single-use and disposable are technically interchangeable, some components designed for single use may see repeated or extended use before their disposal. For example, air-filter capsules are often used over multiple cycles or for several weeks continuously. The number of cycles may be limited by the number of sterilization cycles possible, the rate of filter blockage that occurs, and a change in products to be manufactured — or simply standard change-out practices for a given process. Ultimately, such components must be assessed for waste disposal in the same way as strictly single-use products. We incorporated such extended-use capability into our model system when making the waste disposal assessments shown below.
Other disposable components in the model system require stainless steel hardware for support: e.g., large tangential-flow filtration cassettes and depth filter capsules. However, such hardware has either no fluid contact or very restricted contact and thus does not require the extensive cleaning procedures and high liquid-disposal demands of traditional equivalents.
When comparing the amounts of solid waste in single-use and traditional systems, it is also important to note that although the stainless hardware of traditional systems may be cleanable and reusable, other components contained within it may be single-use in nature. For example, many liquid cartridge filters involved in prefiltration, sterilization, and virus removal are single-use and will contribute to the solid waste generated by traditional systems. We incorporated this consideration into our comparative assessments.
Waste Management Options
Detailed information has been published on waste management of disposable products to assist users in developing their own specific processes (7,8,9). The options available are recycling, incineration, and landfills. Recycling may involve reuse of components, reprocessing of recycled materials, and/or production of liquid fuel by pyrolysis of waste. Incineration can provide energy recovery or not. Landfills are an option for both hazardous and nonhazardous wastes, and landfill-sourced methane may be used to generate power.
The route selected for disposing of used process components is often determined by a company’s procedures for other solid waste already in place. For example, incineration or landfill are still widely used and may be the only practical options in some cases due to constraints on available facilities or the nature of bioharzardous fluids. Also, small-scale disposable systems may not generate sufficient waste to justify material segregation for more environmentally friendly options such as recycling.
Those historic practices may require reassessment as both the scale and extent of disposables increase in a manufacturing plant. In fact, this presents an opportunity to investigate alternative procedures: for example, sorting waste into separate material groups for recycling and reprocessing. Recycling is now standard for much domestic and industrial waste and is assisted by the use of international material identity codes for recycling (10).
Essential Component Information
When we try to apply the above practices to disposable bioprocess components, the task becomes more difficult due to their complexity and/or the presence of more than one material of construction. In this context, component manufacturers and system suppliers can play an important role by supplying detailed and essential information relevant to waste management of their products. However, a simple qualitative list of materials of construction may be insufficient; quantitative data are also important. If more than one type of plastic is present, then it is essential to know the composition of subcomponents and whether they are easily separable after use. Such detailed information, which may not always be available in published product information, can substantially affect the options available for waste management.
To demonstrate how such assessments may work in practice, we broke down our model disposable system into its individual components and their major subcomponents. Table 1 provides the essential information on each item. The data show several features of importance for waste disposal:
Some components (e.g., liquid prefilters and flexible tubing) contain only one polymer, whereas others contain a mixture of polymers. These differences affect the waste disposal options available.
Components such as sterile connectors contain items with different materials of construction, but some are easily separable for waste-disposal purposes, improving the viability of options such as recycling and reprocessing.
In addition to the weight of solid waste, the volume may also be a critical factor. Sometimes it can be reduced substantially by shredding, grinding, compression, or heating.
Table 1: Technical information on selected components of the model single-use system
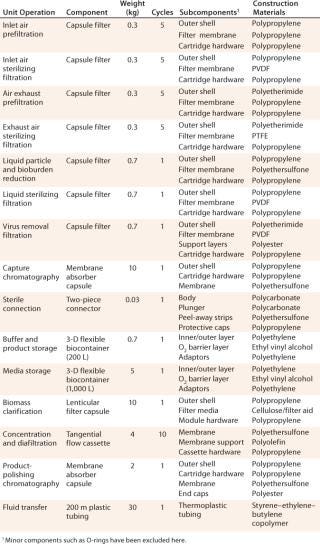
From that detailed information in Table 1, together with a full inventory of disposable components within the system, we calculated the total solid waste for each component type in a model production cycle. Similarly, the solid waste from the disposable elements of a traditional stainless steel system can also be calculated. In Table 2, we compare the solid waste from five unit operations involving each type of system.
Table 2: Comparison of solid waste from single-use and stainless steel systems
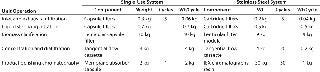
For the components listed in Table 2, it can be concluded that the amount of solid waste per production cycle for each unit operation is, as expected, higher in the single-use system. However, the stainless steel system also produces a significant quantity of solid waste. Other components such as plastic containers and flexible tubing in single-use systems will contribute significantly. And liquid waste for the stainless system will be substantially greater. This simple comparison shows that when a company introduces single-use systems and assesses their impact on solid waste disposal, comparative data for both systems can be vital.
The total amount of solid waste for a specific process depends heavily on the nature of the unit operations involved, the number of components used, and the scale of the process. A typical single-use system for MAb production could use up to 90 bags and biocontainers ranging from 1 L to 1,000 L in size. Because the model system presented here is not a fully detailed process, we could not perform a meaningful calculation of total waste for it. In a comparable study by Sinclair et al., however, based on a model MAb single-use process at a scale of 3 × 2,000 L, the total process solid waste was calculated as 880 kg per batch.
Prescriptive Approach to Waste Management
Together with a list of available waste-disposal options, the data from Table 1 can be used to prepare a prescriptive matrix for individual components. Table 3 provides our results from doing so.
Table 3: Waste disposal options for specific disposable components
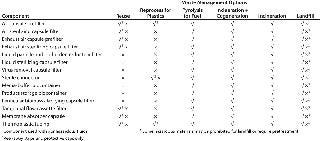
For solid waste that is not suitable for reprocessing, cogeneration (energy recovery from incineration) may be considered. The heat value of plastics is ∼1.5× higher than coal and nearly 3× higher than wood, which makes plastics ideal for energy conversion and produces very little ash. Based on the average heat values for mixed plastics, this solid waste would have an energy value of ∼13 kWh/kg. An additional environmental benefit of this approach is the very low level of emissions and precise control of effluent quality in advanced cogeneration plants.The major limitation on recycling of plastics for reprocessing is the presence of heterogeneous materials of construction, although in some cases the subcomponents of certain items may be separable after use and then recycled. As recycling processes develop, some degree of heterogeneity of waste may be acceptable for reprocessing, which would be of benefit for many disposable components used in biomanufacturing as well as many other industries. Redesign of some components may also be possible to create homogenous waste if it does not degrade functionality.
An alternative recovery process is pyrolysis (producing liquid fuel from plastics), which involves high-temperature heating of plastics in the absence of oxygen, followed by condensation and refining of the resulting hydrocarbons. For mixed plastic wastes, about a liter of liquid fuel is obtained per kilogram of plastic.
Current application of these two developing technologies may depend heavily on other factors, such as the availability of facilities and logistics for transportation of waste. However, such advanced technologies offer great potential for improving the management of biomanufacturing waste for the future and further reducing the environmental impact of single-use components.
A Balanced Approach
The increasing complexity of disposable systems and components presents challenging demands on their users in assessing disposal of the solid waste they produce. Current practices for other waste materials may be applicable, at least in the short term, but are likely to come under increasing scrutiny regarding environmental impact as time goes by. At the same time, the use of traditional stainless steel systems must also be assessed environmentally, especially focusing on the management of liquid waste (water and chemicals) and energy consumption.
Using a model system, we demonstrate that with sufficient essential data on individual components, it is possible to identify suitable waste disposal options. From such a technical matrix, the preferred method can be prescribed for each component with regard not only to practical and financial requirements but, most important, to environmental sustainability. The possibility of using recycling or recovery processes always should be considered. This comprehensive approach to waste management can help ensure minimal environmental impact of single-use processes while providing bioprocessors with the proven benefits they offer.
REFERENCES
1.) Langer, E. 2008.Fifth Annual Report and Survey of Biopharmaceutical Manufacturing Capacity and Production, BioPlan Associates Inc., Rockville.
2.) Fuller, M, and H. Pora. 2008. Introducing Disposable Systems into Biomanufacturing. BioProcess Int. 6:30-36.
3.) Haughney, H, and J. Hutchinson. 2004. Single-Use Systems Reduce Production Timelines. Gen. Eng. News www.hyclone.com/pdf/bp_single_use24gen8.pdf 24.
4.) Foulon, A. 2008. Using Disposables in an Antibody Production Process. BioProcess Int. 6:12-18.
5.) Sinclair, A. 2008. The Environmental Impact of Disposables Technologies. BioPharm Int. http://biopharminternational.findpharma.com/biopharm/article/articleDetail.jsp?id=566014&pageID=1&sk=&date= supplement.
6.) Pora, H, and B. Rawlings. 2009. Managing Solid Waste from Single-Use Systems in Biopharmaceutical Manufacturing. BioProcess Int. 7:18-25.
7.) Wells, B. 2007. Guide to Disposal of Single-Use Bioprocess Systems. BioProcess Int. 6:S24-27.
8.) 2000. Directive 2000/76/EC of the European Parliament and of the Council of 4 December 2000 on the Incineration of Waste. Off. J. Europ. Communities http://eur-lex.europa.eu/LexUriServ/LexUriServ.do?uri=OJ:L:2000:332:0091:0111:EN:PD:91-111.
9.) 2008. Guide for Industrial Waste Management, US Environmental Protection Agency, Washington.
10.) Resin Identification Codes American Chemistry Council.
You May Also Like