Cell Therapy Bioprocessing Technologies and Indicators of Technological ConvergenceCell Therapy Bioprocessing Technologies and Indicators of Technological Convergence
The cell therapy industry is undergoing a natural evolution from scientific curiosity into a commercially and clinically attractive opportunity (1). This evolution is by no means complete, and growing evidence suggests that its progression is driving significant developments in cell therapy bioprocessing — notably, convergence.
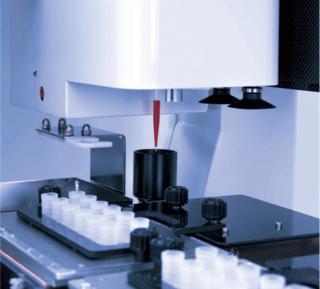
Table 1: 194; ()
Progressively, bioprocessing technologies primarily used in production of noncell-based products are being evaluated for cell therapy bioprocessing applications (2). Consequently, this process of convergence is leading to an increasing proportion of the total portfolio of such bioprocessing options involving technologies that have previously been used in other biomanufacturing applications — such as production of recombinant proteins.
Technological convergence is not unique to cell therapy biomanufacturing (Table 1), or indeed to bioprocessing (Tables 2 and 3). This process shares similarities with technology repurposing; however, the key distinction is that convergence describes the tendency for heterogeneous technological systems to evolve toward performing similar tasks. One example is the convergence of digital audio, data and video tools. Similarly, the trinity of process engineering, commercial considerations, and regulatory requirements drives the convergence of cell therapy bioprocessing technologies.
Table 1:
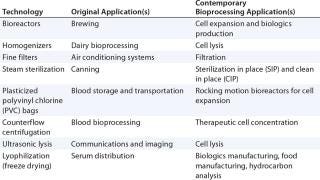
Table 1: 194; ()
Table 2:

Table 2: 194; ()
Table 3:

Table 3: 194; ()
Fundamentally, bioprocess engineering encompasses the design, operation, control, and optimization of biochemical processes to efficiently produce a range of products at requisite scales. This remit is achieved through application of intellectual and physical tools, the selection of which is driven by unique properties of the bioprocess materials involved. In cell therapy bioprocessing, the primary influence is the high sensitivity of cells to their bioprocess environment (3,4).
Unique Characteristics of Cells as a Bioprocessing Material
In production of existing biologics such as monoclonal antibodies (MAbs), cells are used as an expression system that secretes target therapeutic products into their culture media unless products are retained within them and removed through mechanical and/or chemical means. At some point in the downstream biomanufacturing process of such biologics, the interdependence of a cellular expression system’s critical quality attributes (CQAs) and those of the therapeutic product can be separated from one another (5,6). Critically, however, that is not possible with cell therapies because the cells themselves are the product that will be administered (6). Thus, end-product CQAs and the bioprocess environment experienced by therapeutic cells are interdependent and require concomitant optimization, control, and monitoring. The situation applies to not only cell culture operations, but also the full manufacturing process from entry of raw materials through final product formulation.
Industrial cell lines typically used to produce biologics exhibit substantially more physicochemical stability during bioprocessing than “biologically dynamic” therapeutic cells, whose fate is dependent upon their bioprocessing environment. For example, many therapeutic cells exhibit a high degree of sensitivity to their environment, such as
complex nutritional demands
dependence on exogenous growth factors
responsiveness to endogenously produced growth factors
differential behaviors in response to varying pH, dissolved oxygen (DO), and osmolality
responsiveness to mechanical forces
Those limitations may be surpassed by the discovery of molecules and/or biomaterials that can manipulate intracellular signaling and the cells’ microenvironment (9) — or by coculturing cells with biocompatible functionalized materials (8). However, the list of unique demands contribute to limitations in the direct transferability of existing bioprocessing technologies to use in cell therapy bioprocessing.
Existing cell-expansion technologies, for example, frequently require significant adaptations of cells to accommodate their increased sensitivity to environmental stress (e.g., mechanical forces and reactive oxygen species) and to provide appropriate control over differentiation outcomes and maximize differentiation efficiencies. Nevertheless, existing technologies and know-how from the biopharmaceutical industry can revolutionize cell therapy bioprocessing by facilitating integrated process monitoring and control, informing media development and feed optimization, providing cost-effective means for
scaling up or out, and ultimately helping to industrialize cell therapy manufacture. Conversely, the needs of cell therapy bioprocessing are transforming existing technologies with sophisticated monitoring and control approaches, an increasing need for scale-out solutions, and a heavy focus on integrated single-use technologies for implementation throughout the entire manufacturing process. Ultimately, the previously disparate fields of biologics production and cell therapy manufacturing will converge upon more sophisticated and highly controlled bioprocesses.
Although it is apparent that a wide range of bioprocess technologies are available for cell culture operations (Figure 1), options for downstream processing of cell therapy products are currently limited. This perhaps reflects the current stage of industry development, which has so far focused on simply growing sufficient cells to meet clinical trial demands — without substantial investment in optimization of the entire manufacturing process (including downstream processing). Furthermore, it is during downstream processing that typical biopharmaceutical processes diverge sharply from cell therapy because they remove cells as by-products. However, much of the knowledge and technologies involved in biopharmaceutical downstream processing by biopharma can be applicable to cell therapies.
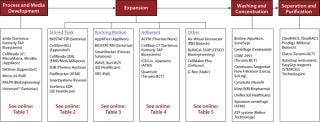
Figure 1: ()
Ideal Characteristics of Cell Therapy Bioprocess Technologies
At all process scales, it is essential that the bioprocess solutions selected not only meet the specific demands of manufacturing cells as products, but also satisfy the regulatory, commercial (demand and cost of goods, CoGs), and infrastructural requirements of the cell therapy industry (10). The relative importance and burden of those challenges is likely to vary throughout product development. For example, academic developers are likely to be constrained by physical and financial resources, so they will place greater emphasis on capital and operating expenditures (11). Conversely, industrialists will focus on regulatory compliance — driving demands for in-line process analytics — and commercial drivers, including CoGs at commercial scales and material-supply risks involved when bespoke consumables are required.
Regardless of scale and target-product characteristics, a number of process technology features are desirable for ensuring robustness of bioprocesses with maximum flexibility and amenability to short-term manufacturing demands and long-term product development strategies (12). These include minimization or (preferably) elimination of manual operations; operator ease; (functionally) closed systems wherever possible; single-use components; scalability (up and out); ease of technology transfer between manufacturing sites; regulatory compliance; a range of in-line process analytical technologies; ease of process optimization; and high-productivity with maximum process efficiency.
A key differentiating feature among cell therapy bioprocessing technologies is their relative automation capabilities. In the academic environment, automation is of limited utility and only a limited number of systems will be in use simultaneously. But industrialization of autologous cell therapies will lead to creation of “bioreactor farms,” where operators must handle dozens or even hundreds of bioreactors at a time — one for each patient — followed by separate downstream processing flows for each patient lot.
Effective cell therapy bioprocess automation also requires enhanced process-control capabilities to enable effective material and patient sample tracking; scheduling optimization; and execution of patient-specific processing protocols (if applicable). Those essential features typically extend beyond the automation regime implemented in conventional biologics production process automation. Additionally, from a risk-management perspective, effective bioprocess automation should reduce patient lot mishandling, including interpatient contamination risks.
Concise Review: Methodology, Implications, and Caveats
We compiled a list of key cell therapy bioprocessing technologies through expert-user interviews; reviews of trade and industry literature; and discussions with technology manufacturers. Thereafter, we obtained technical specifications from publicly available documents and (whenever possible) confirmed them with the technology manufacturers (see Acknowledments)to ensure their accuracy.
It was not possible in all cases, however, to make contact with some manufacturers for corroborating publicly available data or obtaining complete data for all technology features sought. Additionally, our data presented are agnostic to performance for particular cell types, are restricted to single-use technologies, and do not reflect the impact of operator skill and/or regulatory restrictions on technology performance. Due to the significant technological heterogeneity in downstream processing technologies, our discussion is restricted to upstream bioprocess technologies concerning cell culture process and media development. We also omitted the impact of cell characterization and testing technologies that may be used alongside those technologies discussed.
Collation of these data clearly demonstrates the convergence of “traditional” biopharmaceutical technologies with the emerging cellular therapies industry. High-level technical specifications are not always easily obtainable. Technology manufacturers are hesitant to definitively state typical operating ranges for reasons of commercial confidentiality. Potential inter–cell-type idiosyncrasies can also make a difference, as well as as-yet limited user experiences and optimization case studies in which cells are the product. In five electronic-only tables accompanying this article online (www.bioprocessintl.com/bpiextra), we provide an overview of the most common technologies for rapid and straightforward comparison of available options to guide readers’ identification of technologies for further investigation. Online Table 1 details process and media development options listed in Figure 1. Online Table 2 details stirred-tank cell expansion options listed in Figure 1. Online Table 3 details rocking-motion cell expansion options listed in Figure 1. Online Table 4 details adherent-cell expansion options listed in Figure 1. And online Table 5 details other cell expansion options listed in Figure 1.
Process and Media Development
For this analysis, we combined technology options for both process and media development into a single subgroup. However, although some technologies may be suitable for both functions, others will not be. For example, it is likely that the Pall Micro system is suitable for media development but not for process development because of its small working volume. Similarly, Univessel (Sartorius) and/or Celligen (Eppendorf) systems are likely to have excessively large working volumes for most media-development operations. The key differentiator among technologies for process development and media-development operations is probably the design space for final production-re
actor volumes in terms of classical process parameters: mixing time, pH, DO, volumetric power input, and so on. In particular, a large process design space probably is more advantageous for allogeneic (rather than autologous) therapies that necessitate larger process volumes.
The importance of cell culture media on cell CQAs and process performance follows from heterogeneity in the physiochemical properties of individual cell lines (13,14) and the close relationship between the cell bioprocessing environment and cell fate (Figure 2). Consequently, significant efforts are used in cell line specific media optimization both specific and nonspecific to cell lines in cell therapy bioprocessing.
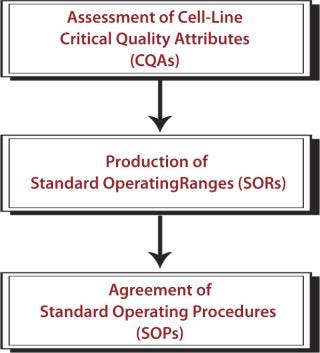
Figure 2: ()
Process and media developments that are not cell-line specific focus on achieving regulatory compliance for early stage technologies, at which point a disparity in expectations exists between academia and industry. For example, to mitigate supply and contamination risks, it is essential to ensure that all process and product components are xeno-free wherever possible. That necessitates removal of xeno-cell feeder layers that have been used to provide physicochemical support to certain cell types as well as removal of animal-derived sera in favor of defined media (15,16).
Therefore, the key differentiators among technologies available to support process and media development are the working-volume range; the number of vessels that can be used in parallel (in some cases each with alternative reaction conditions); the range of “off-the-shelf” and standard components (including impeller types); and the extent of liquid-handling automation. Wherever possible, automated liquid-handling functions are preferred for maximizing throughput and minimizing interoperator variability. Furthermore, process control and in-line analytics are critical to development of robust data sets that support process development and regulatory compliance.
Cell Expansion
For this review, we restricted our consideration of cell-expansion technologies to single-use systems only. This constraint reflects market trends that are driven in part by the desire to minimize contamination risks, ease regulatory compliance, maximize flexibility, and accommodate unique challenges posed by recipient-specific manufacturing strategies (17). Of all unit operations we investigated, it is evident that expansion technologies have been subject to the greatest research and development (R&D) efforts for cell therapy applications thus far, having the most significant body of user experience as well.
Despite significant differences in operating principles and construction, the four main types of expansion technologies we assessed share similar features and specifications, as delineated in the “Value Differentiators” box. Expansion technology choices are principally driven by cell-type attributes (e.g., adherent or nonadherent cells) (18), and the stage of product development (e.g., low capacity and ease of operation for early stages or high capacity and robustness for late stages).
Similar to process and media development technologies, provision of in-line process analytics is critical to cell expansion technologies. It is particularly true for autologous therapy biomanufacturing, in which variable starting-material volumes and CQAs must be accommodated in manufacturing consistent products. Additionally, a range of gas and liquid lines is important for process monitoring and control (19). This may be challenging for proprietary expansion technologies with operational characteristics that can vary substantially to conventional stirred-tank reactors (STRs) hindering performance comparison and evaluation.
One of the most critical properties of single-use cell-expansion systems (as well as single-use media storage and preparation technologies) is the material choice for the disposable components. If selected inappropriately, they can pose a risk of releasing potentially toxic or inhibitory substances. Such leachables are chemical compounds, namely additives to polymers used to manufacture bags and/or other substances generated during gamma irradiation and storage. Hammond and coworkers (20) identified such a compound that can be present at impermissibly high amounts in some single-use bags currently offered in the marketplace. Leachable compounds can migrate from plastic materials to cell culture media — and consequently to cells in culture. The release of cytotoxic compounds under process conditions is particularly undesirable because such chemicals negatively affect cell growth and viability (21).
For cell therapy applications in which downstream processing steps are fairly limited, it is important to prevent cytotoxic effects arising from leachables and extractables. Therefore, it is essential to select suppliers who can understand, specify, and control additive formulations in their disposables as well as in their manufacturing processes (22) to secure patient safety.
One neutral source for such evaluations is a pair of recently published round-robin experiments by the Dechema (23). In these interlaboratory trials involving several large biopharmaceutical companies, bags from all major suppliers were subjected to media and water-for-injection (WFI) extraction followed by a standardized cell culture trial to assess growth. Several bags showed impaired growth compared with controls; others reproducibly supported cell growth without any restrictions. We encourage every scientist using single-use products in the field of cell therapy to enter into a discussion with his or her suppliers on the performance of their respective products during these trials.
Increasing growth for cell bioprocessing technologies is currently met by a combination of cell-specific and non–cell-specific technologies adapted from other industries (particularly biologics manufacturing). It is likely that the latter technologies, representing convergence, will continue until the unique physicochemical demands of cells contained in process fluids necessitate significant adaptions to existing (if not entirely new) technologies. Developments in downstream cell bioprocessing lag behind those in upstream bioprocessing, where the engineering community has sequentially approached production bottlenecks.
We hope that this clear overview of commercially available cell therapy bioprocessing technologies — particularly the online materials —provides a useful resource for both technology users and developers. It highlights implications of the important trend of technological convergence. For readers of the classics, we pose the parting question of, “To what extent does technology repurposing and adaptation represent a Theseusian Paradox?” (24).
Key Value Differentiators in Expansion Technologies
Dependency on other technologies (e.g., microcarriers)
Capital expenditure
Productivity per unit footprint
Monitoring and control capabilities
Rheological
properties (e.g., shear forces generated)
Amenability to a range of cell types
Interbatch consistency
Construction materials (rig and reactor)
Range of working volumes available
Expansion modalities (e.g., batch or fed-batch operation)
Compatibility with “generic” consumables
Ease of sterilization including minimization of “process downtime”
Graphical user interface (ease of operation)
(Functionally) closed
Data “audit trail” for use in regulatory compliance and multiparametric process optimization
Operational costs
Ease of multisite comparability
Efficient gaseous exchange
Heat monitoring and exchange
Potential for application of “artificial intelligence” and/or dynamic feedback in bioprocess control
Ease of maintenance
About the Author
Author Details
David A. Brindley is a researcher in the Nuffield Department of Orthopaedics Rhuematology and Musculoskeletal Sciences at the University of Oxford and the Oxford–UCL Centre for the Advancement of Sustainable Medical Innovation (CASMI), both at the University of Oxford (Oxford, UK); in the Harvard Stem Cell Institute (Cambridge, MA); and in the Centre for Behavioral Medicine at the UCL School of Pharmacy, University College London (London, UK). Anna L. French is consortium manager of CASMI. Ricardo Baptista is a project manager and scientist, and Nick Timmins is director of cell manufacturing at the Centre for Commercialization of Regenerative Medicine (CCRM) in Toronto, ON, Canada. Thorsten Adams is head of product management for single-use bioreactors at Sartorius Stedim Biotech GmbH in Goettingen, Germany. Ivan Wall is a lecturer in regenerative medicine bioprocessing in the Department of Biochemical Engineering at University College London (London, UK). Kim Bure is director of cell therapy at TAP Biosystems (now Sartorius Stedim Biotech GmbH) in Royston, Hertfordshire, UK.
REFERENCES
1.) Daley, GQ, and DT Scadden. 2008. Prospects for Stem Cell-Based Therapy. Cell 132:544-548.
2.) Brandenberger, R. 2011. Cell Therapy Bioprocessing. BioProcess Int. 9:S30-S37.
3.) Brindley, D. 2011. Bioprocess Forces and Their Impact on Cell Behavior: Implications for Bone Regeneration Therapy. J. Tiss. Eng. 00:620247.
4.) Kirouac, DC, and PW Zandstra. 2008. The Systematic Production of Cells for Cell Therapies. Cell Stem Cell 3:369-381.
5.) Berkowitz, SA. 2012. Analytical Tools for Characterizing Biopharmaceuticals and the Implications for Biosimilars. Nat. Rev. Drug. Discov. 11:527-540.
6.) Carmen, J. 2012. Developing Assays to Address Identity, Potency, Purity and Safety: Cell Characterization in Cell Therapy Process Development. Regen. Med. 7:85-100.
7.) Costa, P, FV Almeida, and JT Connelly. 2012. Biophysical Signals Controlling Cell Fate Decisions: How Do Stem Cells Really Feel?. Int. J. Biochem. Cell Biol. 44:2233-2237.
8.) Zhang, H. 2011. Biomimetic Three- Dimensional Microenvironment for Controlling Stem Cell Fate. Interface Focus 1:792-803.
9.) Pashuck, ET, and MM Stevens. 2012. Designing Regenerative Biomaterial Therapies for the Clinic. Sci. Transl. Med. 4:160sr4.
10.) McCall, MA, and DJ Williams. 2013. Developing Cell Therapies: Enabling Cost Prediction By Value Systems Modeling to Manage Developmental Risk. J. Comm. Biotechnol. 19:27-39.
11.) Brindley, DA, IB Wall, and KE Bure. 2013. Automation of Cell Therapy Biomanufacturing: Minimizing Regulatory Risks and Maximizing Return on Investment. BioProcess Int. 11:S42-S49.
12.) Thomas, RJ, PC Hourd, and DJ Williams. 2008. Cell Culture Automation and Quality Engineering: A Necessary Partnership to Develop Optimized Manufacturing Processes for Cell-Based Therapies. J. Lab. Automation 13:152-158.
13.) Pistollato, F. 2012. Standardization of Pluripotent Stem Cell Cultures for Toxicity Testing. Expert Opin. Drug Metab. Toxicol. 8:239-257.
14.) Place, ES, ND Evans, and MM Stevens. 2009. Complexity in Biomaterials for Tissue Engineering. Nat. Mater. 8:457-470.
15.) Cahan, P, and GQ Daley. 2013. Origins and Implications of Pluripotent Stem Cell Variability and Heterogeneity. Nat. Rev. Mol. Cell Biol. 14:357-368.
16.) Lindroos, B. 2009. Serum-Free, Xeno-Free Culture Media Maintain the Proliferation Rate and Multipotentiality of Adipose Stem Cells In Vitro. Cytother. 11:958-972.
17.) Dang, SM. 2004. Controlled, Scalable Embryonic Stem Cell Differentiation Culture. Stem Cells 22:275-282.
18.) Frauenschuh, S. 2007. A Microcarrier-Based Cultivation System for Expansion of Primary Mesenchymal Stem Cells. Biotechnol. Prog. 23:187-193.
19.) Hambor, J. 2012. Bioreactor Design and Bioprocess Controls for Industrialized Cell Processing: Bioengineering Strategies and Platform Technologies. BioProcess Int. 10:22-33.
20.) Hammond, M. 2013. Identification of a Leachable Compound Detrimental to Cell Growth in Single-Use Bioprocess Containers. PDA J. Pharm. Sci. and Tech. 67:123-134.
21.) Wood, J. 2013. Strategy for Selecting Disposable Bags for Cell Culture Media Applications Based on a Root-Cause Investigation. Biotechnol. Progr. 29:1535-1549.
22.) Jurkiewicz, Manuscript in preparation.
23.) Steiger, N, and R Eibl. 2013. Interlaboratory Test for Detection of Cytotoxic Leachables Arising from Single-Use Bags. Chem. Ing. Tech. 85:26-28.
24.) Harding, T 2013. Theseus’s Paradox. The Logical Place yandoo.wordpress.com/2013/08/17/theseuss-paradox.
You May Also Like