Medium and Process Optimization for High Yield, High Density Suspension Cultures: From Low Throughput Spinner Flasks to High Throughput Millilitre Reactors
The most important contributions to high-yield manufacturing processes for the production of recombinant proteins from cultivated mammalian cells have come from the identification of highly enriched and well-balanced media formulations, and fine tuning the process conditions that support high cell culture densities with high specific productivity. The industry standard yield for immunoglobulins or similar molecules derived from suspension-cultivated mammalian cells in bioreactors has risen during the past 20 years from the tens of mg/L to g/L. The more favourable conditions used today for high-yield, large-scale protein expression have typically been identified in small-scale bioreactor systems. Using these “scale-down” systems, attempts were made to match the physicochemical constraints, as closely as possible, to those found in very large bioreactors (up to 25,000 L). But the question remained, how was it possible to establish and use high-throughput, scale-down systems that successfully mimicked such conditions and optimized the hundreds of parameters that interact within a poorly understood network of large cell populations and/or their gas/liquid environment?
The comments presented here attempt to summarize, non-comprehensively, almost three decades of industry observations and development. During the first 20 years, despite the dramatic yield improvements mentioned above, a lack of good scale-down systems resulted in slower than expected progress and contributed to the perpetuated myth that cells in a suspension culture are very difficult to handle. Fortunately, recently identified cell culture systems and the recognition of higher than expected cell robustness in certain media formulations have provided promising new opportunities and have shown that high throughput bioprocesses are, in fact, possible, closely matching large-scale conditions.
Figure 1.
The First Two Decades: Stirred Tanks
Large-scale suspension cultures for the production of recombinant proteins have been used since the early 1980s, with human recombinant tissue plasminogen activator being the first protein made at the 10,000 L scale — using stably transfected and gene amplified Chinese hamster ovary cells (CHO) (1). The cultivation of these cells, which were originally grown in adherent culture, depended on the use of foetal bovine serum (FBS) — up to 20% — in the medium. The emergence of large-scale cultures provided a strong incentive to develop media formulations that initially reduced and then finally eliminated the need for this very expensive additive, the quality of which was, at times, variable and required prior and elaborate testing with respect to its growth-supporting activities.
A most important step towards large-scale suspension cultures was the generation of suspension-adapted subpopulations derived from the adherent populations of cells. Media development and the generation of matching subpopulations for growth in serum-free cultures was done in “spinner flasks,” glass bottles that ranged in volume from 100 mL to 5 L (2,3). However, the workhorse for process development was the 500 mL spinner flask, which was preferably filled with only 150 mL of culture, although volumes of up to 200 mL were possible. The first generation of spinner flasks had a flat Teflon impellor (with an inserted magnetic rod) that was driven by a magnetic stirrer (Figure 1a). The relatively low spin rate of the stirrer (30–50 rpm) ensured a regular, gentle mixing of the suspension culture without the generation of turbulence. It was intentional that these spinner flasks did not contain baffles, in case a high shear stress was exerted on the suspended cells. The filling volume for suspension cultures was generally kept well below the 50% mark, preferably even below 30% of the total volume, to maintain a relatively large headspace over the liquid. This headspace was considered sufficient to supply oxygen to the cells, at least during the initial phases of growth, for CHO cells typically seeded at a density of 200,000–350,000 cells/mL. During the later phases of the culture, when higher densities were expected, the two side-branch access ports of the flask were opened (that is, the caps were opened by one quarter to one half of a turn). Thus, the diffusive entry and exit of gasses to and from the spinner flask occurred along the threading of the cap. With larger working volumes, the bottle had to be opened earlier. Eventually, air sparging through a single open pipe, inserted via one of the side-branch ports, was done to increase oxygen transfer. However, this was found to be quite tricky: critical and high pH values were frequently observed, in a non-reproducible way, as the growth rates in the larger vessels were not very predictable. Thus, the interplay between cell-produced CO2 and its stripping rate via gas bubbling was difficult to predict.
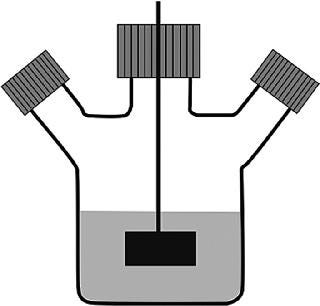
Figure 1. ()
Despite these and other technical problems with glass bottles (washing, sterilization and silanization to prevent wetting and to reduce the attachment of dead cells to the glass), the relatively economical spinner flasks (“non-instrumented” bioreactors) were the only reasonably reliable vessel system that provided data that could be used to predict how bioprocesses would occur in larger cultivation systems. The other main scale-down system was the instrumented bioreactor, whose price tag per vessel, even at the 1–2 L scale, was 50 times that of a single spinner flask. In addition, the set-up and use of fully equipped small-scale stirred reactors was far more time consuming than the preparation and use of spinner flasks. Placing the spinner flasks into a 37 °C warm room or an incubator ensured the proper culture temperature and mixing. Each spinner accommodated up to 4 flasks. In a single warm room, available to one of the authors (FW), up to 40 spinner flasks were used at once to identify optimal process conditions. More recently, a disposable spinner flask, modified with respect to its stirring device and aeration system, the “SuperSpinner” from Sartorius Stedim Biotech (Figure 1b), has an impellor comprising a gas-permeable tube wound around a holder. The advantage of this spinner flask, besides its disposability, is the ability to provide air/oxygen/CO2 mixtures to increase the pH stability and oxygen supply of the culture.
Figure 2.
SuperSpinner D1000, Sartorius stedim Biotech
The most problematic aspects of these low-throughput scale-down systems are listed below. For these reasons, the development and rapid improvement of culture conditions and media formulations were severely held back:
Experiments were hampered by a large degree of variability from one vessel to the next, even when attempts were made to set up everything as close to identical as possible. In the authors’ laboratory, triplicate cultures were generally used so that at least two spinners would show similar trends, in the event that erroneous results would exclude one or two spinners.
One operator running more than 30 spinners was not feasible, both in terms of the generation of the seed cultures (large volumes) and workload.
The limited oxygen supply in the spinners was not recognized for a long time; the insertion of fully instrumented oxygen probes was not done, simply owing to cost, set-up time and sterility problems.
The low throughput rate only allowed an approximate “optimization” of conditions to be made through consecutive experiments, resulting in enormous workloads for many weeks before reasonable conclusions could be drawn.
The minimum volume of about 100–200 mL of culture medium per vessel resulted in very significant volumes of media, adding dramatically to the costs that had to be invested in this work.
Although attempts were made to work with smaller systems, such as with disposable multiwell plates, the results were also unsatisfactory, mostly because of “edge-effects” and water evaporation (see below). Overall, frustration with “non-instrumented” bioreactors — that is, systems that did not control and/or monitor the two most important physicochemical parameters, oxygen and pH — was widespread in the 1980s and 1990s. It led most companies to invest heavily in fully instrumented bioreactors, in which controlled sparging with air or oxygen and pH adjustments ensured a constant environment for optimal cell growth. The smallest of these had a minimum working volume of about 0.7 L, and the price tag for a single fully controlled unit was around $50,000. This fact alone prevented companies from efficiently optimizing their media and delayed relevant research in those academic laboratories that had a genuine interest in mammalian cell culture-based protein production.
Cell Culture Process Development Since 2000
Having recognized the serious limitations of small-scale systems, two trends developed. First, the complex, fully instrumented lab-level bioreactors were reduced in size and were linked together into “multiplexed” systems. Second, non-instrumented vessel systems were down-scaled, taking the most severe bottleneck — oxygen supply — into consideration while also changing the mixing principle from stirring to shaking. While the smaller instrumented bioreactor systems (6–10 reactors, mounted on a single platform, with somewhat simpler control and monitoring possibilities) were becoming more affordable, they still could not deliver high-throughput results. However, they did make access to stirred-tank technology more widespread. There are now several instrumented systems on the market that simultaneously allow 6–12 independent bioreactors to be run on a single platform.
In 2003–2004, the company BioProcessors developed SimCell technology, a robotized bioreactor system that, for the first time, offered high-throughput cell culture optimization with pH and oxygen control in a µL-scale bioreactor. The SimCell System is fully automated, utilizing software-controlled microfluidics and a range of proprietary in-line sensing technologies. These measurements and control strategies enable feedback control of important factors such as pH and dissolved oxygen concentration. The mixing of cell culture suspensions is ensured by a gas bubble that moves within the reactor while, at the same time, the entire microbioreactor array (6 chambers of 700 µL each) is turned vertically on a wheel. To the authorsí knowledge, this high-throughput microbioreactor system has only been sold to a relatively small number of biotech/pharma companies. Unfortunately, very little data from this instrument has been made available in the public domain. Academic groups are probably not purchasing this instrument because of its cost, several hundreds of thousands of dollars.
When not using spinners, many groups still employ disposable multiwell plates for process and medium development. These plates are used for static or shaken cultures in a standard CO2 incubator. However, a severe limitation of these systems is the loss of water. During run times of 7–14 days, the water loss can be extensive (>50%), even in humidified incubators. A reduction in water content with time will increase the osmolarity of the cell culture medium, which is known to severely affect growth and productivity. Also, the individual wells in a single plate — and across several stacks of multiwell plates — are not homogenous regarding gas exchange; the well covers only allow slow, diffusive gas transfer over rather large distances. It is not, therefore, surprising to learn that the desired equivalence in performance from these very small “Bioreactors” to larger instrumented stirred-tanks has not been claimed in any published record.
Around the beginning of the current decade, a paradigm shift in suspension culture technology occurred when it was recognized that “industrialized” cell lines, such as CHO, NS0 and HEK-293 cells, are in fact not that sensitive to shear stress and other physicochemical factors when cultivated in optimized, serum-free media specifically developed for suspension cultures. Fortunately, such media had become accessible from commercial producers, and affordable for academic institutions, in spite of the fact that they cost several-fold more than classical FBS-dependent media. The availability of these media, together with the need to more rapidly and reliably develop high-yield processes, has resulted in the development of more “robust” approaches to scale-down systems geared towards moderate- to high-throughput applications.
A key invention from one of the co-authors of this article (MDJ) was the “TubeSpin” cultivation vessel (4). Essentially, this “non-instrumented” bioreactor is a modified 50 mL centrifuge tube with a conical bottom (to facilitate the accumulation of wet cell pellets) with a ventilated cap. These tubes have been commercially available (under the trade name Cultiflask 50) for a few years from both TPP (Trasadingen, Switzerland) and Sartorius Stedim Biotech (Aubagne, France). See Figure 2. The 50 mL tubes are designed to be mounted vertically on a shaker platform and are orbitally shaken with a displacement radius of 25 mm at a rate of 150–300 rpm. In a humidified and CO2-controlled incubator shaker (Kühner AG, Birsfelden, Switzerland) up to 350 tubes can be mounted simultaneously. Growth performance data during the initial work with these TubeSpin bioreactors (2000–2001) and more recent computer assisted fluid dynamic (CFD) studies has shown that the physicomechanical impact on cells in these highly agitated systems is, in fact, extremely low — despite the rather high velocities of the liquid in the reactors. The CFD studies showed that the liquid layers move as laminar sheets and that stress forces are at least 1.5 logs lower than those required to induce cell damage. We also know from experiments using very high cell densities (>30 million cells/mL) in 30 mL suspension cultures (70% fill volume) that the oxygen supply through the ventilated cap is entirely sufficient (Xiaowei and De Jesus, et al., paper in preparation). The rapid movement of the tube pushes liquid into the headspace, subsequently “washing” the small surface area of the filter inserted into the cap. Any variation in oxygen concentration across the membrane is equilibrated very quickly as the diffusion distance from the outside (100% oxygen relative to air) to the inside is only the thickness of the filter membrane in the cap. Also, because the incubator shaker is humidified, very little water loss is observed with time. Experiments in a 37 °C warm room with no humidification showed that only 30 µL of water was lost per day when only the smallest hole (the D hole) was left open (Figure 2).
Optimization programmes are extremely dependent on scale-down systems that match the performance of cultures in larger vessels.
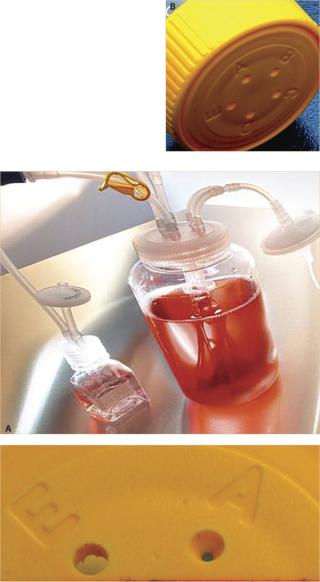
Figure 2. ()
The successful application of TubeSpin technology in process development has now been reported at several cell culture conferences (personal communications and posters). The co-authors have published results showing that up to 1000 TubeSpin bioreactors can be run in a single experiment (5). Another system being used by many groups who discovered the higher-than-expected robustness of industrialized cell lines in good media is the “classic” Erlenmeyer flask. For cell culture applications, disposable, cap-ventilated bottles are available and can be mounted on an orbital shaker in a humidified incubator. The 250 mL flask with a cell culture volume of 50–100 mL is a widely used workhorse (Dr Martin Jordan, Merck-Serono, personal communication). It is the authors’ opinion that oxygen supply in these flasks remains a limiting factor, because the orbitally shaken fluid will probably not engage with the air in the headspace to the same extent as it does in TubeSpin bioreactors. The narrowing of the bottle neck most likely reduces the air movement in the upper part of the flask, creating a “quiet” zone that makes oxygen transfer in the headspace less efficient. Although Erlenmeyer flasks are widely used in the industry, few details have been published on oxygen transfer and cell performance in these vessels.
In Conclusion
The standard volumetric yields (at least for antibodies) of multiple grams per litre from mammalian cell cultures have resulted from decades of investigation into cells, media and processes, and have been obtained by the diligent efforts of hundreds of technicians, engineers and scientists who have worked on high-yield manufacturing processes based on two or three cell lines (CHO, NS0 and, more recently PER C6 cells). The key contribution has not been the vector, the transcriptional promoter/enhancer or the specific productivity; instead, the dramatically improved cell density, maintained at high viabilities for 2–3 weeks using sophisticated nutrient feeds and process steps (temperature, pH, osmolarity shifts, etc.), has accounted for most of the increased productivity. The development of media to support these higher cell densities and the identification of fast growing, robust cell populations was mostly done in instrumented 1–3 L stirred-tank bioreactors, through iterative sets of experiments taking several months. We believe that such high-yield processes could have been obtained much earlier if more effort had been geared towards the establishment of simpler scale-down systems. Unfortunately, popular misconceptions dictated the need for very sophisticated control and process conditions to obtain good reactor-based growth and productivity. As has been shown very recently, a simple, slightly modified centrifuge tube, when shaken vigorously, can support the very high cell densities needed to get the multigram yields mentioned above. These tubes do not have direct pH control and they are not sparged. Instead, they have a very high gas-transfer rate to supply oxygen via headspace aeration (with air). Their performance equivalence to stirred-tank bioreactors is based on the fact that the cells in these tubes never run out of oxygen. There are still a number of issues with TubeSpin technology that need to be better understood and/or characterized; however, these vessels have shown themselves to be very useful in our hands and are now considered to be routine instruments in many cell culture-based optimization programmes, ranging from transfections in suspension to biomaterials testing with fibrous polymers (E. Engelhard, et al., in preparation).
Optimization programmes are extremely dependent on scale-down systems that match the performance of cultures in larger vessels — because of the high number of interacting media components and the interplay between process control and process steering parameters. Design of experiment (DOE) approaches had been useless in the past because the difference between the physiology of cells in poor scale-down models and the “real” conditions in large-scale bioreactors was just too great. Now, it is hoped, that an emerging understanding of small-scale systems that mimic large-scale vessels will provide unprecedented opportunities for further improvements in cell performance in biotech applications.
REFERENCES
1.) Wurm, FM. 2004. Production of Recombinant Protein Therapeutics in Cultivated Mammalian Cells. Nature Biotech. 22:1393-1398.
2.) Griffiths, B 1986.“Scaling Up of Animal Cell Cultures”Animal Cell Culture, IRL Press, Oxford:33-69.
3.) Scharfenberg, K, and R Wagner. 1995.A Reliable Strategy for the Achievement of Cell Lines Growing in Protein-Free MediumAnimal Cell Technology: Developments Towards the 21st Century, Springer, Dordrecht:619-623.
4.) De Jesus, MJ. 2004. TubeSpin Satellites: A Fast Track Approach for Process Development with Animal Cells Using Shaking Technology. Biochem. Eng. J. 17:217-223.
5.) Stettler, M. 2007.1000 Non-Instrumented Bioreactors in a WeekCell Technology for Cell Products, Springer, Dordrecht:489-495.
You May Also Like